用于储能应用的氧化铁纳米结构的进展
摘要
日常生活中对绿色高效储能设备的需求不断上升,这是由全球环境和能源问题引起的。锂离子电池(LIBs)是一种重要的储能装置,备受关注。石墨被用作LIBs负极,但其理论容量较低,因此有必要开发更高容量的LIBs负极。本文综述了近年来新型氧化铁及其复合材料作为锂离子电池负极的应用策略和研究进展。在此我们列举了几种典型的合成方法来获得多种氧化铁基纳米结构,如气相沉积、共沉淀、电化学法等。用于表征氧化铁基纳米结构,特别是原位 X 射线衍射和 57 详细阐述了 Fe Mössbauer 光谱。此外,还讨论和总结了基于氧化铁的纳米结构及其复合材料的电化学应用。
图形摘要
<图片>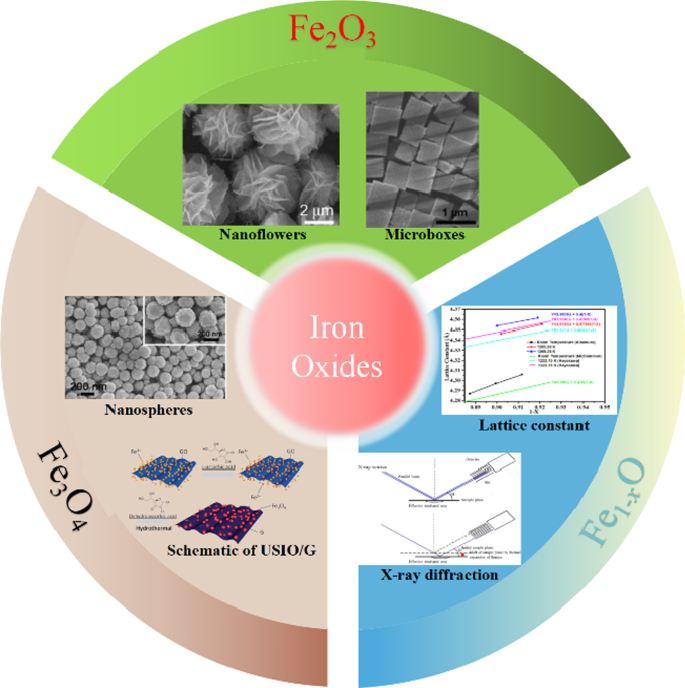
介绍
全球能源和环境问题导致对高效绿色能源的需求不断增加,例如太阳能、燃料电池、锂离子电池(LIBs)和热电模块等[1,2,3,4,5,6, 7,8,9,10,11]。研发高性能、低成本的储能系统是解决这些问题的重要途径。在应用广泛的储能器件中,LIBs 是高效储能系统的重要候选者 [12,13,14,15,16,17,18,19,20,21,22,23,24]。然而,目前商业石墨负极存在局限性,例如理论容量相对较低(372 mAh g −1 ),一些电化学活性材料被提议应用于 LIBs [25,26,27,28,29]。 2000 年,Poizot 等人。 [30] 报道称,过渡金属氧化物 (TMO) 被认为是 LIB 中一种重要的阳极,因为它们的理论容量是石墨的 2-3 倍。因此,TMOs 和相关复合材料作为 LIBs 阳极备受关注。在锂的嵌入/脱嵌过程中,TMOs 发生以下反应[31]。
$${\text{M}}_{x} {\text{O}}_{y} + 2y{\text{e}}^{ - } + 2y{\text{Li}}^{ + } \leftrightarrow x{\text{M}}^{0} + y{\text{Li}}_{2} {\text{O}}$$ (1)其中,M代表Ni、Cu、Fe、Co等。在锂嵌入过程中,这些氧化物被锂还原,形成由分散在非晶Li2O基体中的金属簇组成的复合物[30, 31]。
在 TMO 中,基于氧化铁的负极是一种在 LIB 中具有巨大潜力的优秀候选材料,因为它们具有丰富、低成本和无毒等优点[32, 33]。然而,与其他 TMO 类似,用作 LIB 阳极的氧化铁有两个关键问题。一是较大的不可逆容量,由第一次放电过程中电解质分解和固体电解质界面(SEI)层的形成引起。此外,Fe 和 Li2O 的形成在热力学上是可行的,锂离子 (Li + ) 来自 Li2O 是热力学不稳定的 [34],因为一部分 Li + 无法从第一次放电过程中形成的 Li2O 中提取。这也导致部分不可逆容量。另一个问题是它们的循环稳定性低,主要是由于在 Li + 的插入/脱嵌过程中体积变化大和 Fe 严重聚集。 ,导致电极粉化和容量快速衰减 [3]。为了解决这些问题,很多努力都集中在克服这些问题上,并提出了一些非常有效的方法。据我们所知,一种高效的策略是氧化铁的纳米结构 [3, 35]。对于一些独特的纳米结构,应变和体积变化是由 Li + 的插入/脱嵌引起的 将在很大程度上受到抑制,Li + 可以很容易地在电极中扩散,从而显着提高阳极的电化学性能[11]。此外,还引入了碳质材料,例如碳纤维 (CFs)、碳纳米管 (CNTs)、石墨烯和热解碳等,用于与氧化铁复合 [36,37,38]。这些具有独特结构的碳质材料可以缓冲复合电极在充放电过程中的体积变化,从而增加氧化铁纳米结构的电子接触和循环稳定性。
在这篇综述中,最近关于氧化铁(Fe1−x 阐述和总结了基于 O、Fe2O3、Fe3O4) 的纳米结构在 LIB 和超级电容器中的应用。具体而言,我们专注于基于氧化铁的纳米结构的合成和设计,以及它们的电化学性能。
Wustite (Fe1−x O)
方铁体 (Fe1−x O) 是一种非化学计量化合物,具有 0 <x <0.0464 [11]。它具有岩盐立方结构,其晶格常数为 ~ 4.330 Å [11]。 Fe1−x 与 Fe2O3 和 Fe3O4 相比,O 在储能方面的应用较少,因为它的比容量相对较低,而且低于 843.15 K 的亚稳相容易分解为 Fe 和 Fe3O4。然而,Fe1−x O是一种非常有前景的锂离子电池负极,其电导率高于Fe2O3和Fe3O4。
合成和表征
据我们所知,具有高性能覆盖的 TMOs 的复合对于高性能 LIBs 阳极具有巨大的潜力[7]。在LIBs中,维氏体阳极的电化学机理描述为以下方程[8]。
$${\text{FeO}} + 2{\text{Li}}^{ + } + 2{\text{e}}^{ - } \leftrightarrow {\text{Fe}} + {\text{Li }}_{2} {\text{O }}$$ (2)Gao 等人使用简便的方法合成了 FeO/C 复合材料。 [31]。在合成过程中,将尺寸为 30-120 nm 的 α-Fe2O3 颗粒与不同百分比的乙炔黑 (AB) 混合,然后通过球磨获得均匀的混合物。然后,将 α-Fe2O3 和 AB 的混合物在 N2 气氛中在 800°C 下碳热还原 10 小时,以获得均匀的 FeO/C 复合材料。 FeO/C 复合材料比 Fe2O3/AB 混合物具有更高的循环稳定性。当AB含量为50 wt.%时,FeO/C复合材料的容量为511 mAh g -1 , 高于 396 mA h g −1 Fe2O3/AB。此外,50次循环后的容量保持率> 96%,明显比Fe2O3/AB高70-80%。 FeO/C复合材料优异的电化学性能应归功于其较高的电导率,这是由于碳热还原后FeO和AB颗粒之间的连接增强所致。
此后,在 2016 年,Jung 等人。 [11] 通过使用多元醇还原将 K 热扩散到 Fe2O3/石墨烯中,制备了钾 (K)-Fe3O/石墨烯复合材料作为基于掺钾 Fe3O 纳米颗粒的 LIBs 阳极。通过煅烧 K 掺杂的 Fe2O3/石墨烯,将菱面体 Fe2O3 晶体转化为 FeO 晶体(面心立方,FCC),显示出(111)晶面的宽 d 间距(5.2 Å)。与之前研究的 Fe2O3/石墨烯复合材料 [11] 相比,K-FeO/石墨烯的放电容量为 1776 mA h g -1 在 100 mA g −1 的电流密度下,在 50 次循环中具有高循环稳定性 ,而 Fe2O3/石墨烯的放电容量为 1569 mAh g −1 .即使在 18.56 A g -1 的高电流密度下 , K-FeO/石墨烯的容量保持在851 mAh g -1 800 次循环后。当电极在 18.56 A g -1 的高电流密度下循环更长的时间后,这种差异要大得多 .如图1所示,与Fe2O3/石墨烯相比,K-FeO/石墨烯阳极具有独特的晶体结构和反应机理。 K-FeO/石墨烯的高放电容量表明通过储存额外的Li + 应该是由于钾扩散到 Fe2O3 晶格中而产生的 Wustite 晶格内的空位和宽 d 间距。
<图片>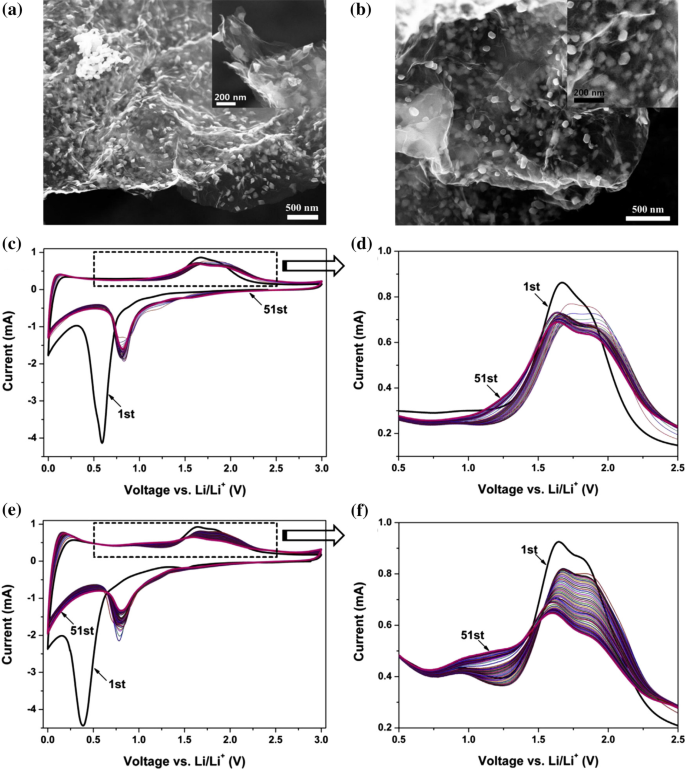
经 [11] 许可转载。版权所有,Elsevier B.V
a 的 SEM 图像 Fe2O3/石墨烯和b K-FeO/石墨烯,以及 (a , b ) 分别是它们的放大图像。 c 的重复循环伏安图 , d Fe2O3/石墨烯电极和e , f K-FeO/石墨烯电极。以 0.5 mV s -1 的电位扫描速率连续扫描两个电极第 51 个循环 .
图>原位 X 射线衍射
通过原位X射线衍射(XRD),可以在短时间内获得样品反应过程中实时结构变化的信息,以及大量的可比信息。它不仅可以在合成过程中观察样品的结构变化,还可以用来检测样品在不同温度下充放电到一定电位下的相应结构变化,对监测实际反应机理非常有用电池中的阳极/阴极。此外,我们小组研究了维氏体晶格常数的精确知识,这是研究其在高温下的物理和化学性质所必需的。 Fe1−x O 合成并通过高温 X 射线衍射仪(RINT2000-TTR,Rigaku Denki Co., Ltd.)与平行光束(图 2a)进行表征,用于测量特定的衍射峰,以及其组成与晶格常数之间的关系还研究了高温方氏体。以 α-Fe (95 wt.%) 和 Fe3O4 (99 wt%) 粉末为起始原料合成方铁矿的过程是共析反应(图 2b)。该反应可以在 843.15 和 1673.15 K 之间在一定 Pco/Pco2 下进行,因为方氏体在 843.15 K 以下不稳定,反应物为 19% Fe (wt.%) 和 81% Fe3O4 (wt.%)。图 2c 显示了初始反应物(磁铁矿和铁)的 XRD 图。实验条件如表 1 所示。 吹扫氦 (He) 气后 (25 ml min -1 ) 进入 XRD 系统的炉子中 60 分钟,反应物以 10 °C min -1 的恒定速率加热 直到样品温度达到 843.15 K,同样在 He 气氛中,流速为 25 ml min -1 .然后排出He气,将CO/CO2气体按一定比例(如1:1和1:2)吹入炉内。通过熔化金片校准高温后,可以获得样品架的实际温度。从 843.15 K 到所需温度(1265.28 和 1365.28 K),升温速率为 2 °C min -1 并采用 XRD 测量样品以确认维氏体相。当样品在所需温度下保存且大部分为方氏体相时,在 240-420 min 内测量方氏体晶面的衍射角,然后将样品温度升高 50 °C 并保持在该温度60 分钟。完成这些步骤后,样品温度降低到原先的所需温度,并在 180-240 min 内再次进行 XRD 测量方氏体晶面的衍射角。
<图片>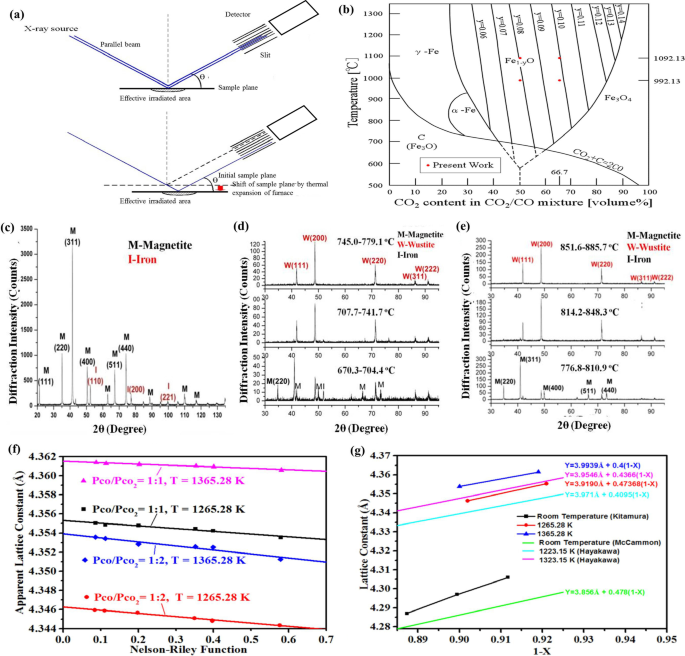
一 原位XRD示意图。 b Fe, Fe1−y 之间的平衡 O、Fe3O4、CO、CO2 和碳。 c 初始反应物的 XRD 图。 d 在 Pco/Pco2 =1:1 下合成的样品的 XRD 图。 e 在Pco/Pco2 =1:2下合成的样品的XRD图谱。 f 在不同温度和 Pco/Pco2 下合成的方铁矿的不同 NR 函数-表观晶格常数。 g 方氏体晶格常数结果
图> 图>晶格常数可以通过将表观晶格常数线性外推到该函数的零来获得,即 2 Theta =180°。方铁体的(111)、(200)、(220)、(311)和(222)晶面的衍射峰如图2d、e所示,样品的XRD图在Pco/Pco2下获得分别为 1:1 和 1:2。获得了不同 Pco/Pco2 和温度下表观晶格常数与 Nelson-Riley 函数之间的关系,如图 2f 所示。直线代表拟合数据的方块。通过这些直线,将表观晶格常数外推到 Nelson-Riley 函数的零。因此,如图2f所示:在不同温度和Pco/Pco2下得到的真实晶格常数的结果为4.355 Å(1265.28 K,Pco/Pco2 =1:1),4.346 Å(1265.28 K,Pco/Pco2 =1:2)、4.362 Å (1365.28 K, Pco/Pco2 =1:1) 和 4.354 Å (1365.28 K, Pco/Pco2 =1:2)。如图2g所示,晶格常数随着x的增加而增加 Fe1−x O,温度越高,晶格常数越大。高温下方氏体的成分和晶格常数之间的关系可以得到如下方程。 (3)和(4)。
$${\text{a}}\,\left( { {\AA} } \right) =3.919 + 0.474\left( {1 - x} \right){ }\left( {1265.28\,{\text { K}}} \right)$$ (3) $${\text{a}}\,\left( { {\AA} } \right) =3.994 + 0.400\left( {1 - x} \right ){ }\left( {1365.28\,{\text{ K}}} \right)$$ (4)57 铁穆斯堡尔光谱
57 Fe Mössbauer 光谱涉及原子核的特性,包括原子核的能级结构和原子核所在的化学环境。因此,它可以相应地应用于研究原子的化合价、化学键的离子性、配位数、晶体结构、电子密度和样品的磁性。 57 Fe Mössbauer 光谱学广泛应用于化学和材料领域。在此,我们详细阐述了 57 Fe Mössbauer 光谱用于表征氧化铁。 57 Fe Mössbauer光谱用于区分和表征各种氧化铁相,并监测晶格中Fe原子的局部环境[39, 40]。
超精细参数,如异构体位移 (IS)、四极分裂 (QS)、四极位移 (ɛ Q) 和超精细磁场 (B hf) 可以通过分析穆斯堡尔谱 [41, 42] 中谱线的位置来获得。从谱线的宽度和不对称性可以推断出样品的特性。通过超精细参数的温度和场依赖性也可以推导出有价值的参数。
奥尔登等人。 [42] 研究了 Fe1−x 的锂诱导转化反应 O 使用 57 Fe Mössbauer 光谱。超精细参数(IS 和 QS)在反铁磁性(TN =198 K [43])Fe1−x 中是 FeII 物种的相当特征 O,在室温 (RT) 下显示出典型的顺磁吸收。如 57 中所示 Fe Mössbauer 光谱(图 3a),有三个加宽的双峰,IS ~ 1 mm s -1 和 QS 范围从 ~ 0.50 到 1.50 mm s −1 , 并且它们的相对面积从 α-Fe 磁贡献校正。吸收强度为 42、26 和 15%。第四个双峰以 IS ~ 0.55 mm s −1 为中心 , QS ~ 0.90 mm s −1 FeII I 的相对面积为 11% 物种,正如预期的非化学计量 Fe1−x O. 来自 FeII/FeII I 比率,空位量估计为 ~ 0.057 ± 0.008,通过XRD表征接近0.050。最后,双峰位于 QS ~ 1.68 mm s −1 和 IS ~ 0 mm s −1 ,对应于α-Fe,贡献 ~ 6%。
<图片>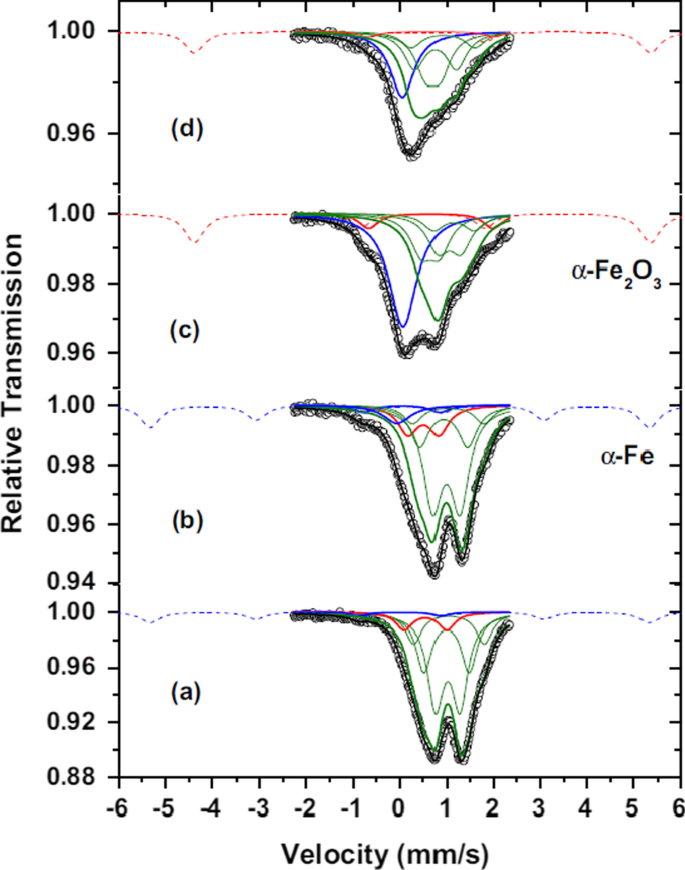
经 [42] 许可转载。版权所有,Elsevier Masson SAS
57 的比较 原始 Fe1−x 在 300 K 下的 Fe Mössbauer 光谱 O 材料 (a ),在吸收 1 Li (b ) 并在 2.16 Li (c )。绿色贡献归功于 FeII。较粗的绿线是与未反应的 Fe1−x 对应的较细绿线的总和 O. 蓝色虚线对应于 (a ) 到 (c )。在 (c ),磁性六重奏已经作为眼睛的指南稍微移动了。频谱 (d ) 对应于 0.94 Li 的第一次充电结束,没有 α-Fe 的贡献,但蓝色单线态纳米金属 ε-Fe 0 仍然存在。
图>Fe1−x 与 Fe2O3 和 Fe3O4 的比容量比较 O 最低。此外,其合成方法较为复杂。大多数情况下,高温还原反应是不可避免的[3,4,5,6,9,11]。结果,Fe1−x 与Fe2O3或Fe3O4相比,O不是理想的LIBs阳极。
Fe2O3 基纳米结构
在这些氧化铁中,尤其是Fe2O3,由于理论容量高达1000 mAh g -1 而引起了众多研究人员的关注。 [44]。此外,Fe2O3 具有独特的优势,如高耐腐蚀性、低生产成本、环境友好、不易燃、无毒和高天然可用性 [45]。由于这些优异的性能,Fe2O3 在 LIBs 负极中的应用非常有前景 [46,47,48,49,50]。在此,简要概述了 Fe2O3 基纳米结构的合成、表征和电化学性能的最新进展。
合成与表征
在过去的十年中,人们在探索 Fe2O3 基纳米结构的合成方法方面付出了巨大的努力。在本节中,我们阐述和总结了 Fe2O3 基纳米结构的合成方法,包括气相沉积法 [51]、溶液法 [52]、电化学法 [53]、热处理 [54] 和其他方法 [55、56]。此外,我们还比较了不同的合成方法。
此外, 57 详细描述了 Fe2O3 纳米结构的 Fe Mössbauer 光谱表征。由于只有某些原子核有共振吸收, 57 Fe Mössbauer 光谱不受其他元素的干扰。 57 的范围 Fe穆斯堡尔谱受核外环境影响一般在几个纳米范围内,非常适合表征纳米结构。
气相沉积
气相沉积广泛应用于合成许多薄膜和其他纳米结构,例如 Fe2O3 和其他基于氧化铁的纳米结构。化学气相沉积(CVD)、原子层沉积(ALD)、物理气相沉积(PVD)、电解沉积和反应溅射是气相沉积的典型方法[57,58,59,60]。
ALD 是一种独特的合成高结晶度薄膜的方法,也是比液相沉积更便宜的途径。在ALD工艺中,每一层的化学反应都直接伴随着前一层。这样,每个反应循环仅沉积一层。例如,林等人。 [51] 利用 ALD 在 TiSi2 纳米网上沉积高质量的超薄 α-Fe2O3 薄膜。 3D 自组织纳米多孔薄膜由 Yang 等人制造。 [61] 通过 CVD 并集成到异质 Fe2O3/Fe3C-石墨烯中。作为LIBs阳极,通过沉积这种薄膜可以大大提高其倍率容量和循环性能。塞萨尔等人。 [62] 通过常压 CVD (APCVD) 沉积了掺硅 Fe2O3 树枝状纳米结构的薄膜,这产生了 Fe2O3 光阳极,可在可见光下以前所未有的效率氧化水。树枝状α-Fe2O3纳米结构的宏观表面积为0.5 cm 2 [62]。 Wu 等人通过金属有机 CVD (MOCVD) 在硅衬底上生长垂直排列的 α-Fe2O3 纳米棒阵列。 [63]。更重要的是,贾等人。 [64]利用射频溅射沉积制备α-Fe2O3超薄膜。
虽然气相沉积能够制备高质量的 Fe2O3 基纳米结构,但它也有缺点。例如APCVD和MOCVD在前驱体过程中具有高毒性和可燃性。
基于解的合成方法
基于溶液的合成方法是常见且容易制造 Fe2O3 和其他氧化铁的方法。具有各种形态的 Fe2O3,例如纳米花 [65]、纳米球 [66]、纳米粒子 [67]、纳米棒 [68]、纳米管 [14]、纳米环 [69]、纳米带 [70]、纳米薄片 [71]、纳米线 [72] ]、纳米纤维 [73] 和微盒 [54] 是通过基于溶液的方法合成的,例如水热法、溶剂热法和溶胶-凝胶法。这些方法非常容易和可用。钟等人。 [65] 使用溶剂热法通过乙二醇介导的自组装过程制造 Fe2O3 纳米花(图 4)。 Vayssieres 等人。 [52] 报道了通过水热工艺在掺氟氧化锡 (FTO) 导电玻璃上生长多孔 Fe2O3 纳米棒阵列。通过 α-Fe2O3 前驱体在 SnO2 纳米线茎上的水热生长,合成了一种新的六重对称分支 α-Fe2O3/SnO2 异质结构(图 5)[74]。还有另一种简便且经济的技术,即溶胶-凝胶法,用于合成 Fe2O3 纳米结构。吴等人。 [68]利用溶胶-凝胶法通过无处不在的Fe 3+ 反应获得α-Fe2O3纳米棒 在反胶束中。通过该机制获得的纳米棒具有低维数和高表面积,可扩展到磁铁矿和方铁矿。
<图片>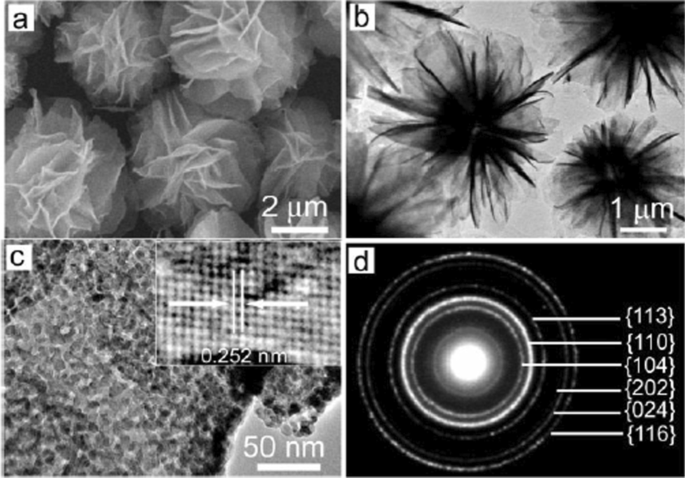
经 [65] 许可转载。版权所有,Wiley-VCH
一 SEM 和 b 所获得的 α-Fe2O3 的 TEM 图像。 c 所获得的 α-Fe2O3 花状结构花瓣的高倍 TEM 图像。 d 所得α-Fe2O3的SAED图。
图> <图片>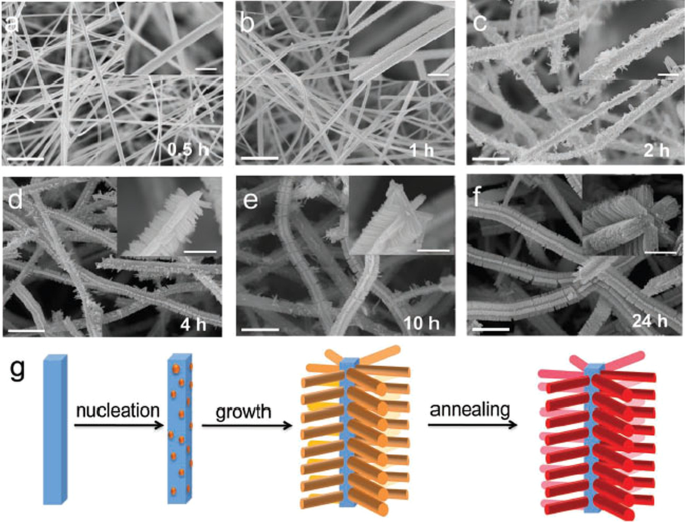
经 [74] 许可转载。版权所有,Wiley-VCH
一 –f 通过设置反应时间,产品(退火后)在不同反应阶段的 SEM 图像。插图是相应的放大 SEM 图像。图中和插图中的比例尺分别为 2 μm 和 500 nm。 g 分层组装的α-Fe2O3/SnO2纳米复合材料的形成过程示意图。
图>电化学法
电化学方法用于合成 Fe2O3 纳米结构,例如,电化学沉积应用于制造 Fe2O3 纳米颗粒 [53]。通过在含有去离子 (DI) 水和 NH4F 的乙二醇电解液中以 30-60 V 的电压对铁箔进行阳极氧化,获得了 α-Fe2O3 纳米管阵列 [75]。此外,采用电化学阳极氧化来合成 Fe2O3 纳米管阵列 [76]。毛等人。 [77] 报道了使用电化学沉积合成 Fe2O3 阵列。在他们的研究工作中,通过电化学沉积将铁沉积到 AAO 模板通道中,然后通过 NaOH 溶液去除 AAO 模板,最后将铁纳米棒阵列转化为 Fe2O3 阵列。本研究的特点是通过改变沉积时间可以精细控制Fe2O3纳米棒的长度。
热处理
用于合成 Fe2O3 的热处理涉及两种重要的方法,热氧化和热解。例如,张等人。 [54] 通过普鲁士蓝 (PB) 微立方体在 350–650 °C 的热诱导氧化分解制备 Fe2O3 微箱(图 6)。与广泛使用的基于溶液的方法相比,固态方法将为均匀各向异性中空结构的大规模合成提供更简便的方法。通过控制热氧化参数制备了不同形貌的 Fe2O3。提出了通过对铁基前体进行简单热处理来制备 α-Fe2O3 纳米结构。例如,Rao 和 Zheng [71] 利用热处理制备了致密排列的 α-Fe2O3 纳米薄片阵列。
<图片>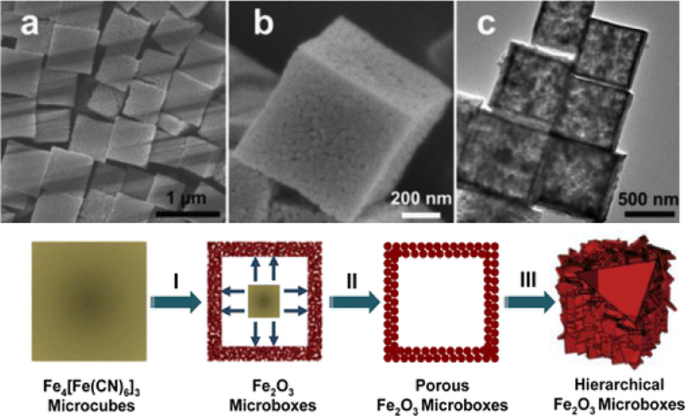
经 [54] 许可转载。版权所有,美国化学会
一 , b FESEM 和 c 在 350 °C 下获得的空心 Fe2O3 微箱的 TEM 图像。 d 随着煅烧温度升高,空心Fe2O3微箱的形成及壳结构演变示意图。
图>热裂解是另一种常见的沉积 Fe2O3 薄膜的方法。杜雷特等人。 [78] 应用这种方法通过超声喷雾热解获得细观 α-Fe2O3 小叶薄膜,而制造的薄膜比传统的喷雾热解技术制造的薄膜具有更高的光活性。此外,还报道了通过气相沉积、液相沉积和热处理合成α-Fe2O3纳米薄片、纳米花、纳米线和纳米棒阵列[63, 71, 72, 79]。
其他方法
2016 年,Guivar 等人。 [55] 复合空位有序磁赤铁矿 (γ-Fe2O3) 纳米颗粒与纳米羟基磷灰石 (nanoHAp) 功能化,使用典型的共沉淀化学途径。值得注意的是,γ-Fe2O3 被纳米HAp 标记为γ-Fe2O3@HAp 是在没有任何热处理过程中形成的。
合成Fe2O3的方法很多,但大多不环保。 2019 年,Bashir 等人。 [56] 开发了一种使用 Persea Americana 种子提取物获得 α-Fe2O3 纳米颗粒的环保方法。他们使用两种不同的前驱体制备了两种 α-Fe2O3 样品,一种样品 (A) 由 Fe(NO3)3·9H2O 制备,另一种样品 (B) 制备 FeCl3·9H2O。
57 在 300 K(室温 (RT))下记录的样品 A 和 B 的 Fe Mössbauer 光谱如图 7 所示。 57 Fe Mössbauer 光谱是一种非常有价值的技术,用于探索特定基质中铁原子的局部磁性行为和氧化态 [80]。两个样品都显示出磁性有序,并且仅显示单个六重奏,表明磁性有序状态。表 2 显示了 57 Fe 在 RT 中提取了穆斯堡尔参数,其中列出了 IS、ɛ Q 和 B 高频。两个样本的B 高于 51 T 的 hf 与 α-Fe2O3 相关 [81]。此外,ɛ的值 Q 也与α-Fe2O3 一致。两种四极杆相互作用都将 Fe 表示为 Fe 3+ 因为观察到的样品 A 和 B 的 IS 分别为 0.3653 mm/s 和 0.3754 mm/s,对于 Fe 3+ 来说是典型的 [82]。因此,四极分裂的负值表明样品A和B的铁磁性较弱,具有纯α-Fe2O3相的特征。
<图片>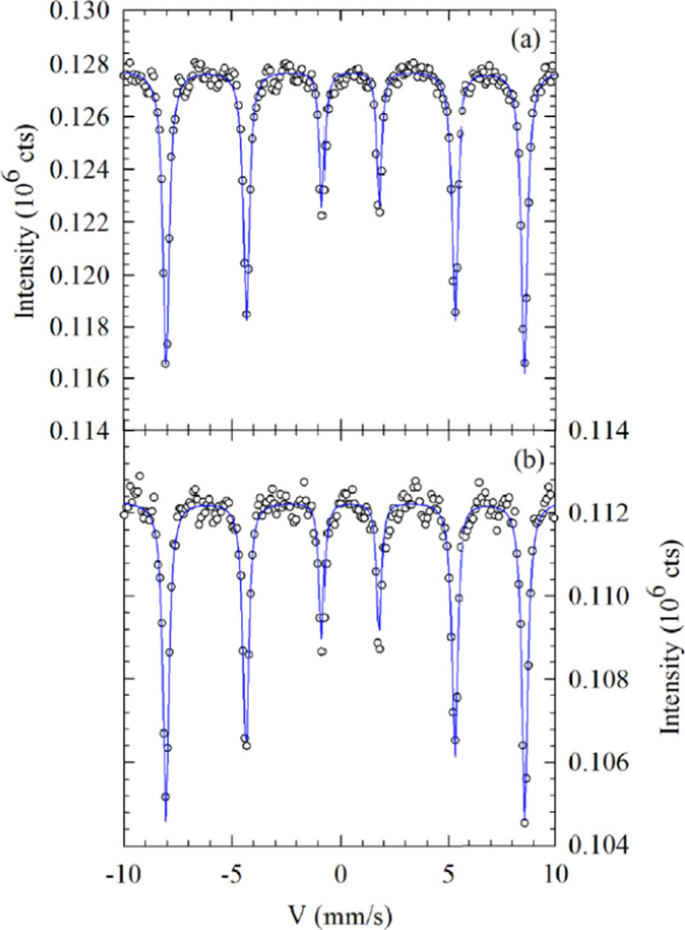
经 [56] 许可转载。版权所有,美国化学会
57 α-Fe2O3 纳米粒子在室温下记录的 Fe Mössbauer 光谱。 一 样品 A(硝酸铁作为前体)和 b sample B (ferric chloride as a precursor).
图> 图>Electrochemical Performance
The charge/discharge cycling at the voltage window of 0.005–3.0 V (vs. Li + ) under a current density of 200 mA g −1 at RT is shown in Fig. 8a. During the initial discharge process, there is an obvious voltage platform of ~ 0.75 V, and it gradually moved to a voltage plateau of ~ 1.0 V, and remain stable in the second and fifth cycles. Meanwhile, an ambiguous plateau was observed at ~ 1.8 V in the charge process. The first discharge profile qualitatively resembles the results by Larcher et al. [83] and Morales et al. [84] on nanoparticle Fe2O3, and Wang et al. [14] on Fe2O3 nanotubes. The cycling performance of three samples (hierarchical Fe2O3 microboxes, Fe2O3 microboxes and porous Fe2O3 microboxes) is discribed in Fig. 8b. After 30 cycles, hierarchical Fe2O3 microboxes exhibit the highest reversible capacity of 945 mA h g −1 , follow by 872 mA h g −1 for porous Fe2O3 microboxes, and finally 802 mA h g −1 for Fe2O3 microboxes. The results demonstrated that three samples display excellent cycling stability, and the morphology of nanostructured Fe2O3 plays a significant role in determining the discharge characteristics.
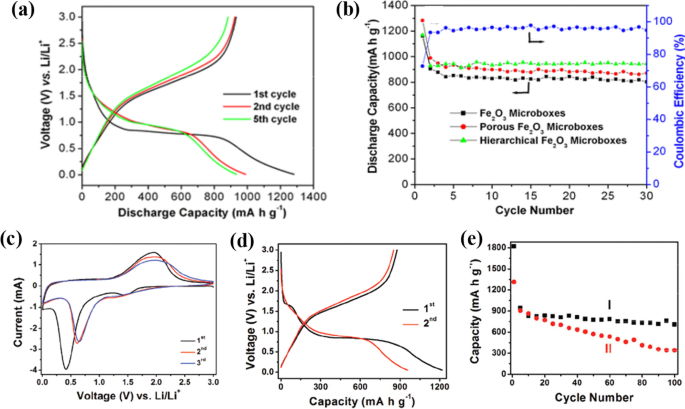
c –e Reprinted with Permission from [85]. Copyright, American Chemical Society
一 Discharge − charge voltage profiles of porous Fe2O3 microboxes obtained at 550 °C. b Cycling performance of Fe2O3 microboxes (350 °C), porous Fe2O3 microboxes (550 °C), and hierarchical Fe2O3 microboxes (650 °C) and Coulombic efficiency of porous Fe2O3 microboxes (550 °C) over the voltage range 0.01 − 3.0 V vs. Li/Li + at the same current density of 200 mA g -1 . (a , b Reprinted with Permission from [54]. Copyright, American Chemical Society). Electrochemical measurements of the sample. c CVs between 5 mV and 3 V at a scan rate of 0.5 mV s −1 . d Charge–discharge voltage profiles. e Comparative cycling performance of (I) the as-prepared α-Fe2O3 hollow spheres and (II) α-Fe2O3 microparticles. All the galvanostatic tests are performed at a constant current rate of 200 mA g −1 between 0.05 and 3 V.
图>Cyclic voltammogram (CV) is used to characterize the cells with α-Fe2O3 nanoflakes anode in the 0.005–3.0 V under a slow scan rate (at RT). Li metal is used as the counter and reference electrodes [20], consistent with previously reported results [85]. CV curves of α-Fe2O3 hollow spheres between 5 mV and 3 V at a scan rate of 5 mV s −1 are presented in Fig. 8c. There are apparent redox current peaks, and demonstrate good reversibility of electrochemical reaction. As shown in Fig. 8d, a distinct voltage plateau can be discovered at ~ 0.75 V, consistent with CV curves. The charge–discharge voltage profiles reflect the lithium storage capacity of α-Fe2O3. The first cyclic discharge capacity and the charge capacity is 1219 mA h g −1 and 877 mA h g −1 respectively, which lead to a relatively low irreversible capacity loss of 28%. In the second cycle, the Coulombic efficiency increased quickly to 89%. Cycling performance of two samples are demonstrated in Fig. 8e. The sample (I) exhibits excellent cyclic capacity retention from the second cycle onward. After 100 cycles of charge/discharge, the reversible capacity is still as high as 710 mA h g −1 . Compared with α-Fe2O3 microparticles, the unique hierarchical α-Fe2O3 hollow spheres apparently have enhanced Li storage performance, and a more stable cycling capacity retention and a higher reversible capacity are realized. This superior performance can be attributed to the thin nanosheet subunits that provide rapid and efficient transport of Li + , as well as the unique hollow interior that allows the material to effectively buffer the stress generated during charge/discharge process.
Iron oxides are cheap, abundant and environmentally compatible, and Fe2O3 has excellent electrochemical performance. Some of the above studies have shown that Fe2O3 based nanostructures can be an alternative anode to replace the presently used graphite in LIBs. The nanostructured Fe2O3 has great potential in LIBs anode [86,87,88,89,90,91,92,93,94,95]. Recently, several studies about Fe2O3 anode in asymmetric supercapacitors are reported [96,97,98,99,100]. However, low surface area and poor electrical conductivity are still two critical issues limiting the specific capacitance and power density of Fe2O3. For solutions of these problems, CNTs and CNFs are regarded as conductive matrices to load Fe2O3 nanoparticles for realizing improved performance [101]. Table 3 summarizes some typical Fe2O3 based nanostructures with their synthesis and electrochemical performance.
图>Fe3O4 Based Nanostructures
Magnetite (Fe3O4) and Fe3O4 based nanostructure composites with pseudocapacitance, high theoretical capacity, environmental friendliness and low cost, are extensively applied to electromagnetic wave absorption [106], LIBs [3, 12, 15], biotechnological devices [107, 108], and supercapacitor [4, 109]. Fe3O4 nanoparticles is one of the high-performance anodes in electrochemical devices. Unfortunately, Fe3O4 nanostructures still face some problems, such as a severe volume variation (~ 200%) during the insertion and extraction of Li + and relatively low electrical conductivity, posing negative influence on the cycling stability [19, 110, 111]. From research results, we found that the structure and morphology of Fe3O4 have a high influence on the electrochemical performance of Fe3O4 and Fe3O4 based composites.
Synthesis and Characterization
Recently, nanostructure engineering is demonstrated as a highly-effective approach to obtain improved electrochemical performance of Fe3O4 and Fe3O4 based composites. Therefore, various nanostructures including 0D nanoparticles [112], 1D nanorods/wires [113, 114], 2D nanoflakes/sheets [115, 116], 3D hierarchical/porous architectures [117, 118], and hybrid nanostructures of iron oxides [16] are proposed. The electrochemical performance of Fe3O4 nanostructures can be optimized by rational design of their morphology, composition, porosity and surface characteristics.
Solution phase synthetic method is a facile and rapid way to obtain Fe3O4 based nanostructures, because of the associative advantages, such as low synthesis temperature (always below 250 °C), easy control of morphology via adjusting hydrothermal conditions (e.g., PH, density of reactant and dosage of active agent, etc.). Solution phase synthetic method includes solvothermal synthesis [119], thermolysis [120], co-precipitation [121], sol–gel process [122, 123], micro-emulsion [124], etc. Simultaneously we compare the pros and cons of these methods.
Solvothermal Synthesis
Solvothermal synthesis, which reacts in a special closed reaction vessel, is one commonly used methods for synthesizing Fe3O4. In a hermetic environment, it is a facile method using aqueous solution as reaction medium at high temperature and high-pressure hermetic environment. Fe3O4 nanostructures with various morphologies (0D, 1D, 2D and 3D) were synthesized applying this approach.
An et al. [119] obtained the Fe3O4/graphene nanowires by solvothermal synthesis and calcination with FeCl3, NH4VO3 and graphene as precursors. Phase transition of Fe3O4/VOx (FVO) after annealing was confirmed by XRD. For the XRD pattern of sample without annealing, all diffraction peaks of FVO and graphene decorated FVO correspond to FeVO4·1.1H2O. For the XRD pattern of sample after annealing, there are no peaks of any vanadium oxides. And the inductive coupled high frequency plasma (ICP) result indicated that the molarity ratio of Fe and V is ~ 0.94:1, confirming the existence of amorphous vanadium oxide.
Mu et al. [125] reported dispersed Fe3O4 nanosheets on carbon nanofiber by combing the electrospinning and solvothermal process. In this work, Fe3O4 nanosheets are uniformly attached on the surface of carbon nanofiber with the diameter of about 500 nm.
Fe3O4 nanoparticle with high specific surface area via FeCl3 and organic solvent ethanolamine (ETA) as precursors is reported by Wang et al. [126]. In this preparation, ETA is critical factor for compounding Fe3O4 nanoparticles with high specific surface area, and Fe 3+ is gradually reduced to Fe 2+ by ETA during dissolution process, demonstrating that Fe 2+ increased as the increase of ultrasonication time. The ratio of ETA and FeCl3 has a large impact on the nanoscale grain size and specific surface area of Fe3O4. And the results showed that the grain size of 20–40 nm is achieved with 60 mL ETA and 6 mmol FeCl3. When the amount of ETA is 80 mL, smaller nanoparticles (5–10 nm) are obtained.
Another representative work is reported by Chen et al. [127], in which graphene nanosheets decorated with Fe3O4 nanoparticles (USIO/G) were synthesized using a facile solvothermal process. For the synthesis of USIO composite decorated with reduced graphene oxide (RGO), is used FeCl3·H2O as precursor, then NaHCO3 and L-ascorbic acid were added to form USIO/G. In this process, L-ascorbic acid was oxidized to dehydroascorbic acid (DHAA) by some of Fe 3+ , which were reduced to Fe 2+ . Formation process of USIO/G is schematically shown in Fig. 9. The Fe3O4 nanoparticles with uniform distribution, which are beneficial for electrical conductivity of graphene, mitigation of volume expansion of Fe3O4, and facilitating Fe3O4 particles into the electrolyte.
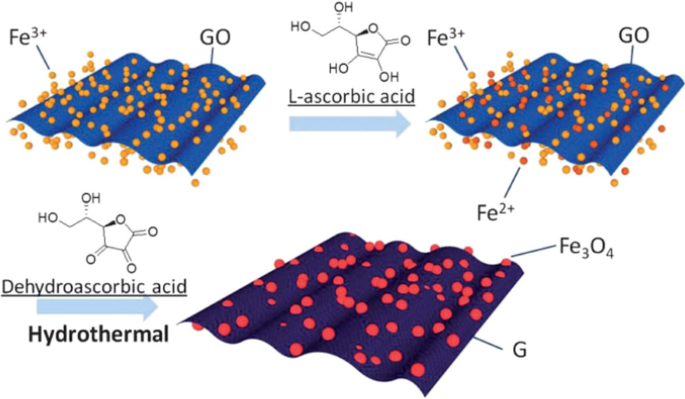
Reprinted with Permission from [127]. Copyright, Royal Society of Chemistry
Schematic illustration of the formation process of USIO/G.
图>Xiong et al. [128] a kind of hierarchical hollow Fe3O4 (H-Fe3O4) microspheres prepared by controlled thermal decomposition of iron alkoxide precursor. In a classical reaction, ethylene glycol (EG) serves as reduction reagent that partly reduces Fe 3+ to Fe 2+ with sodium acetate (NaAc), and polyvinylpyrrolidone (PVP) [128]. For this synthesis, PVP served as a surface stabilizer, which has important role in the formation and transformation of hollow interiors.
With the development of solvothermal synthesis, it emerging as an efficient method with the advantages of low energy consumption, little reunion and easy to control shape, etc. Chen et al. [129] synthesized poly (acrylic acid) (PAA)-entangled Fe3O4 nanospheres by a facile solvothermal method. In their synthesis, the ethylenediamine is crucial to the controlling of the uniformity of nanospheres, and the PAA molecules served as carbon source that transforms into the carbon matrix by heating treatment in inert atmosphere. As shown in SEM image of the prepared C-Fe3O4 nanospheres, very uniform spherical particles with a diameter of 150–200 nm are synthesized. Observed from SEM images in Fig. 10a, the nanospheres contain small irregular particles, and have a relatively rough surface. In the control experiment without ethylenediamine (EDA), the synthesized particles are much less uniform with a wider size distribution of 100–500 nm, allowing the formation of nanospheres with smaller size.
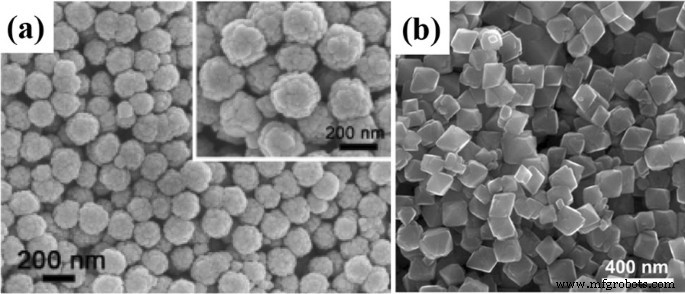
Reprinted with Permission from [130]. Copyright, Wiley–VCH
一 SEM images of the Fe3O4 nanospheres synthesized with ethylenediamine (EDA). The inset of (a ) shows a SEM image with higher magnification. (Reprinted with Permission from [129]. Copyright, American Chemical Society.). Physicochemical characterization of the octahedral Fe3O4 nanoparticles. b SEM image showing the octahedral geometry of the iron oxide nanoparticles.
图>Co-precipitation
Due to its high cost-effectiveness, environmental friendliness, and facile synthesizing protocol, co-precipitation is a general approach for Fe3O4 nanoparticles. Thus, in iron based rechargeable battery systems, Fe3O4 nanomaterials are especially suitable for large-scale electrochemical applications to solve the energy requirement of the modern society.
李等人。 [121] proposed Fe3O4 polyhedron as LIBs anode for alkaline secondary batteries by a co-precipitation. Annealing temperature makes a high effect on the physical and electrochemical performance of Fe3O4 nanomaterials. The 700 °C-annealed Fe3O4 exhibited a higher electrochemical performance, such as a higher specific discharge capacity of 604.2 mA h g −1 with a charging efficiency of 83.9% at 120 mA g −1 . Ooi et al. [130] demonstrated octahedral Fe3O4 nanoparticles using a facile solvothermal route. Scanning electron microscope (SEM) image of Fe3O4 nanoparticles is shown in Fig. 10b, which depicts that octahedral Fe3O4 nanoparticles with an average length of 93 ± 18 nm were prepared by the hydrothermal method, showing a roughly Gaussian size distribution. Then, the crystal structure of octahedral nanoparticles can be further evaluated by HRTEM, and the composition of the bulk sample was further characterized by XRD and X-ray photo electron spectroscopy (XPS).
Thermolysis
The thermolysis is small monodisperse magnetic nanocrystals synthesized by organic metal compounds in high boiling point solvents containing stabilizing agent. Previous Organic metal bodies include metal acetylacetone compounds, metal cupferron, or metal Carbonyl compounds, and usually choose fatty acids, oleic acid, or hexadecyl amine as a surfactant.张等人。 [120] reported ultrafine Fe3O4 nanocrystals uniformly encapsulated in two-dimensional (2D) carbon nanonetworks through thermolysis of Fe(C5H7O2)3 precursor at 350 °C under vacuum, which named as 2D Fe3O4/C nanonetworks. This facile process using low-cost precursor proposed a green approach for preparing Fe3O4/carbon composite. Additionally, compared with the reported Fe3O4/carbon composites, the particle size of Fe3O4 is controllable and a size of ∼ 3 nm can be obtained.
Benefitting from synergistic effects of carbon nanonetworks with excellent electrical conductivity and ultrafine Fe3O4 particles with uniform distribution, high reversible capacity, excellent rate capability and superior cyclability at the voltage of 0.01–3.0 V (vs. Li/Li + ) are obtained. Nanoparticles with unique iron oxide (Fe3O4) cores and zinc oxide (ZnO) shells were prepared by Jaramillo et al. [131]. Fe3O4 nanoparticle synthesized through a thermolysis method using Fe(C5H7O2)3 as organic metal body presoma, triethylene glycol as surface active agent, and core–shell Fe3O4@ZnO nanoparticles were successfully synthesized using straightforward methodologies. The structural and optical properties of the materials were characterized using a combination of X-ray diffraction, electron microscopy, and light spectroscopy. Importantly, the purity of the core and shell phases in the Fe3O4@ZnO nanoparticles was confirmed by both XRD and TEM, and the ZnO shell was shown to increase the transparency of the core–shell nanoparticles relative to the single-component Fe3O4 nanoparticles.张等人。 [132] demonstrated a high crystalline Fe3O4-graphene composite by one-step reaction of thermolysis. And they demonstrated that the attachment of iron-organic complex with graphene oxide (GO) sheets can facilely result in magnetic graphene composites via a time-dependent calcination process.
Sol–Gel Process
The specific method is using the metal alkoxide, metal mineral compound or a mixture of the above two substances to hydrolysis and polymerization, uniform gel gradually, then condense into a transparent gel, however, after drying and heating, finally the oxide ultrafine powders was received. Tang et al. [122] prepared nanostructured magnetite thin film by sol–gel method using inexpensive iron (II) chloride precursor. Fe3O4 nanoparticles were prepared at 300 °C, however, α-Fe2O3 is generated when temperature increased to 350 °C, and this result restricts its applications. Xu et al. [123] proposed magnetite nanoparticles by virtue of sol–gel process combined with annealing in vacuum at 200–400 °C using nontoxic and low-cost ferric nitrate. In their study, Fe3O4 nanoparticles with various sizes can be synthesized facilely through varying the annealing temperature.
Micro-emulsion Method
Micro-emulsion is composed of two mutual miscibility of liquid mixture of thermodynamic stability and isotropy dispersion, one of these or two kinds of liquid called micro area, and fixed by interface layer of the surfactant molecules. The key factors controlling the reaction solution contain concentration, pH value, reaction time and temperature. Micro-emulsion as a rapid expansion of new technology possesses many advantages. For example, high purity and uniform particle size distribution molecular dopant was synthesized at low temperature and simple reaction process. But there are also some shortcomings, for instance, the reaction system mostly contains organic solvents, which leads to high cost, pollution of environmental health and long reaction time. The prepared Fe3O4 nanoparticles have excellent catalytic performance for the synthesis of quinoxaline in different solvents. Novel core–shell magnetic Fe3O4/silica nanocomposites with triblock-copolymer grafted on their surface (Fe3O4@SiO2@MDN) were successfully synthesized by combining sol–gel process with seeded aqueous-phase radical copolymerization approach [133]. The Fe3O4@SiO2@MDN microspheres were synthesized in following three steps. Firstly, the initial magnetic Fe3O4 microspheres were synthesized by a solvothermal reaction. Then a sol–gel process was utilized to prepare silica coated Fe3O4 microspheres (Fe3O4@SiO2), and a thin amorphous silica layer was formed on Fe3O4 microspheres. Afterward, the Fe3O4@SiO2 microspheres were modified by 3-(methacryloxypropyl) trimethoxysilane (MPS). Finally, the triblock copolymer was fabricated by aqueous phase radical copolymerization reaction among MPS, divinylbenzene (DVB) and N-Vinyl-2-pyrrolidone (NVP) on the surface of Fe3O4@SiO2. The magnetic Fe3O4 particles with narrow size distribution have nearly spherical shape and smooth surface.李等人。 [124] reported hexagonal and triangular monodisperse Fe3O4 nanosheets by a two-step microemulsion solvothermal approach, in which the uniform Fe3O4 nanoparticles are prepared and then these hydrophobic nanocrystals are dispersed in a uniform microemulsion environment as “seeds” for further re-growth through a secondary solvothermal process. In the first step, near-spherical monodisperse 7–8 nm Fe3O4 nanoparticles were formed through a kinetically controlled process. In the second step, the formation of anisotropic Fe3O4 nanosheets is a thermodynamically controlled process and all the exposed surfaces of the triangular and hexagonal nanosheets are (111) crystal planes, which have the lowest surface energy for FCC Fe3O4.
Other Methods
Physical methods are also significant ways to prepared Fe3O4 nanostructure for anode of LIBs. Several advantages, such as good crystallization, fine-tuned particle size, and high purity of products are highlighted in recent literatures. But these methods usually demand advanced and expensive equipment, result in a higher cost, poor dispensability of particles dispersion, and agglomeration of nanostructures. For instance, Du et al. [109] fabricated activated carbon (AC)-Fe3O4 nanoparticles asymmetric supercapacitor, and Fe3O4 nanostructure was prepared by microwave method. The precursor, FeSO4·7H2O and NH3·H2O mixed solution, was heated in microwave oven. The black precipitate was separated by magnet and washed repeatedly with DI water. The resulted microstructural properties of prepared nanoparticle were characterized by nitrogen adsorption (Quantachrome NOVA 2000), XRD and SEM [109].陈等人。 [127] synthesized graphene nanosheets decorated with ultra-small Fe3O4 nanoparticles (USIO/G).徐等人。 [134] reported an integrated usage of magnetic particles in microalgal downstream processes, specifically microalgal harvesting and lipid extraction through one-step aerosol spray pyrolysis and applied in microalgal harvesting and serial microalgal lipid entrapment. TEM/EDS, XPS, and FT-IR analysis suggested that the cationic and lipophilic functionalities arose from not fully decomposed PVP, due to the short residence time in the reactor. Kang et al. [135] proposed Fe3O4 nanocrystals confined in mesocellular carbon foam (MSU-F–C) by a “host–guest” approach and applied it as LIBs anode. In this study, a precursor of Fe(NO3)3·9H2O is impregnated in MSU-F–C having uniform cellular pores with a diamter of ~ 30 nm, followed by heating treatment at 400 °C for 4 h in argon (Ar) atmosphere. Fe3O4 nanocrystals with size of 13–27 nm were fabricated inside the pores of MSU-F–C. The existance of the carbon most likely allows the reduction of some Fe 3+ to Fe 2+ ions by a carbothermoreduction process. The physical performance and pore structure of MSU-F–C and Fe3O4-loaded composites were characterized with nitrogen sorption, and the composites have high capacities of ∼800–1000 mA h g −1 at 0.1 A g −1 (∼0.1 C rate), high rate capability and good cycling performance.
Application
Fe3O4 possesses lots of unique properties, and is highly promising for applications in LIBs and supercapacitors [136,137,138,139,140,141,142]. Table 4 summarizes some applications.
图>Li-Ion Batteries
Due to conversion reaction of Fe3O4 during charge/discharge process and other advantages, the Fe3O4 is usually studied and applied as LIBs anode [143,144,145,146,147]. For TMOs, they have higher theoretical capacity (~ 500–1000 mA h g −1 ) than conventional graphite (about 372 mA h g −1 )。 Furthermore, Fe3O4 has superior conductivity compared with other transition metal oxides. Thus, it is well-focused by recent studies. It has been reported that composite electrodes with graphene have high performance due to their large surface area, high electrical conductivity and adaptive or flexible structure for high reliability. Qiu et al. [3] reported a kind of composite anode composed of ultra-dispersed Fe3O4 nanoparticles (3–8 nm) and RGO sheet. It has excellent cyclic performance (624 mA h g −1 for up to 50 charge/discharge cycles at a current density of 0.1 A g −1 ), and good specific capability (624 and 415 mAh g −1 at 0.1 and 2.4 A g −1 , respectively) for LIBs. The obtained Fe3O4/RGO exhibited high and ultrastable Photo-Fenton activity (Fig. 11).
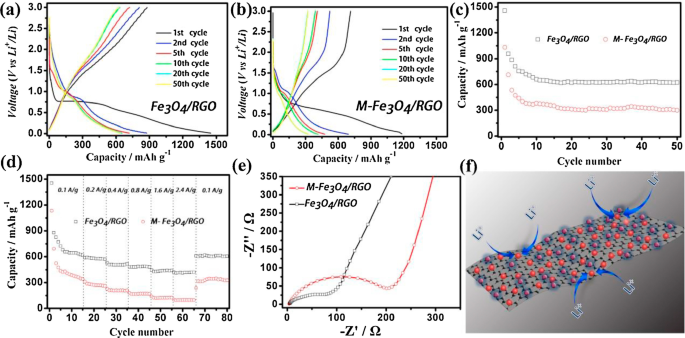
Reprinted with Permission from [3]. Copyright, Elsevier B.V
The charge/discharge curve of Fe3O4/RGO composites (a ) and mechanically mixed Fe3O4/RGO composites (M-Fe3O4/RGO) (b ) electrodes at constant current densities of 0.1 A g −1 . Cycling performance of Fe3O4/RGO composites and M-Fe3O4/RGO composites electrode at constant current densities of 0.1 A g −1 (c )。 Rate capability of Fe3O4/RGO composites and physically mixed Fe3O4/RGO composites at the current densities between 0.1 A g −1 and 2.4 A g −1 (d )。 Nyquist plots of the electrodes of Fe3O4/RGO sheet and mechanically mixed Fe3O4/RGO composites. All of the measurements were conducted using a voltage window of 0.01–3.0 V (e )。 Schematic representation of the electrochemical reaction path on the Fe3O4/RGO composites (f ).
图>Pyrolyzed carbon is also a good “companion” for Fe3O4 anodes. Apart from the facile protocol, porous structure formed by pyrolysis always exhibits high specific capacity of Fe3O4 composite anode.王等人。 [12] reported hollow N-doped Fe3O4/C nanocages with hierarchical porosities by carbonizing polydopamine-coated PB nanocrystals as LIBs anode (Fig. 12). The specific capacity of N-doped Fe3O4/C nanocages is ~ 878.7 mA h g −1 after 200 cycles at a current density of 200 mA g −1 , much higher than that of N-doped Fe3O4/C derived from pure PB (merely 547 mA h g −1 )。 It is also desirable to design anisotropic structure of Fe3O4 nanoparticles with carbon coated layer.张等人。 [19] reported a kind of carbon-coated Fe3O4 nanospindles derived from α-Fe2O3 nanospindles with length of about 500 nm and an axis ratio of ~ 4. Following by a hydrothermal synthesis method with glucose, the obtained LIBs anode delivered a high reversible capacity of ~ 745 mA h g −1 at C/5 and ~ 600 mA h g −1 at C/2.
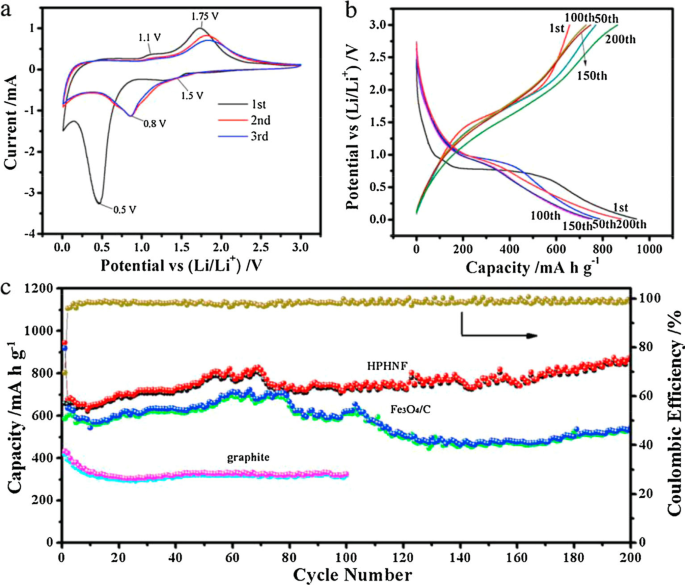
Reprinted with Permission from [12], Copyright, Elsevier Ltd
一 CVs of the HPHNF during the first three cycles at 0.2 mV s −1 , b Galvanostatic charge/discharge profiles of the HPHNF electrodes for the 1st, 50th, 100th, 150th and 200th cycle at a specific current of 200 mA g −1 . c Cycling performance of the HPHNF nanocomposites, N-doped Fe3O4/C nanocomposites and graphite at a specific current of 200 mA g −1 . d Coulombic efficiency of HPHNF.
图>The most impressive work towards this field is probably the mesoporous iron oxide nanoparticle clusters with carbon coating reported by Lee et al. [148]. After a few cycles, the formation of SEI greatly enhanced the stability of interface between electrode and electrolyte. Electrochemical test exhibited a high specific capacity of 970 mA h g −1 for LIBs.
Supercapacitors
Fe3O4 is a highly promising candidate for supercapacitor electrode because of its relatively high electrical conductivity, fast reversible redox reaction, low cost and eco-friendly nature [149,150,151,152]. Similar to batteries, high performance supercapacitors also require two factors:large specific surface area and long-term stability. Those two features usually were achieved by building some porous structures and carbon coated layers. Fe3O4 nanoparticle with a high specific surface area was synthesized by Wang et al. [126] using a bottom up approach. Ferric chloride was firstly sonicated with ethanolamine and then processed through a solvothermal reaction. The obtained active nanomaterials showed a specific surface area of 165.05 m 2 g −1 and a specific capacitance of 207.7 F g −1 at 0.4 A g −1 .
Also, highly dispersed Fe3O4 nanosheets on 1D CNFs is reported by Mu et al. [125]. The Fe3O4/CNFs composites showed a higher specific capacitance than pure Fe3O4 in 1 M Na2SO3. To further enlarge the specific capacitance and cycle stability, hierarchically porous carbon spheres with Fe3O4 using as supercapacitors exhibited high capacitivity of 1153 F g −1 at 2 A g −1 and high specific capacitance of 514 F g −1 at 100 A g −1 . In addition, the assembled asymmetric supercapacitor with double-shelled hollow carbon spheres and Fe3O4, has excellent cycling stability (96.7% retention after 8000 cycles) and high energy density (17–45 Wh kg −1 ) at a power density of 400–8000 W kg −1 [4].
Conclusion
Iron oxides (Fe1−x O, Fe2O3, Fe3O4) based nanostructures have much higher specific capacities than those of commercial carbon based anodes. They are considered as highly promising candidates for LIBs anode. However, large irreversible capacity and low cycle stability are two serious problems that obstruct the application of iron oxides based nanostructures. In this review, we summarized the recent progress on novel iron oxides and their composites as LIBs anode and supercapacitor electrode. Several typical synthetic methods of various novel iron oxides based nanostructures are listed. By comparing the electrochemical performance of these various iron oxides based nanostructures, some strategies are expected to solve the problems of iron oxides based nanostructures.
数据和材料的可用性
Not applicable.
纳米材料