用于癌症治疗的纳米粒子:当前的进展和挑战
摘要
癌症是导致死亡和发病的主要原因之一,具有复杂的病理生理学。传统的癌症疗法包括化学疗法、放射疗法、靶向疗法和免疫疗法。然而,缺乏特异性、细胞毒性和多药耐药性等局限性对有利的癌症治疗构成了重大挑战。纳米技术的出现彻底改变了癌症诊断和治疗领域。纳米粒子(1-100 nm)具有生物相容性、毒性低、稳定性更好、渗透性和滞留效果增强、靶向精准等特殊优势,可用于治疗癌症。纳米粒子分为几个主要类别。纳米颗粒药物递送系统是特殊的,并且利用了肿瘤和肿瘤环境特征。纳米粒子不仅解决了传统癌症治疗的局限性,还克服了多药耐药性。此外,随着新的多药耐药机制的解开和研究,纳米粒子正在得到更积极的研究。纳米制剂的各种治疗意义为癌症治疗创造了全新的视角。然而,大部分研究仅限于体内和体外研究,多年来批准的纳米药物数量并没有太大的放大。本综述讨论了多种类型的纳米粒子、靶向机制和批准的纳米治疗药物在癌症治疗中的肿瘤学意义。此外,我们还总结了当前临床转化的前景、优势和挑战。
介绍
癌症是一组以不受控制的、随机的细胞分裂和侵袭性为特征的疾病的统称。多年来的广泛努力一直集中在检测癌症的各种危险因素上。对于某些癌症,病因与特定环境(后天因素)如辐射和污染有很大关系。然而,不健康的生活方式,如不均衡的饮食、烟草消费、吸烟、压力和缺乏体育锻炼,会强烈影响癌症风险的确定 [1, 2]。虽然这些外部因素已被认为是癌症的主要原因,但原癌基因突变、抑癌基因表达模式和参与 DNA 修复的基因的参与一直难以估计。只有 5-10% 的癌症病例与遗传基因有关 [3]。高龄是癌症和许多个体癌症类型的另一个关键风险因素。
癌症是全球重大的公共卫生问题之一,是导致死亡的第二大原因。据美国癌症协会称,到 2021 年底,新病例数预计将达到 190 万[4]。用于癌症治疗的常规治疗方法包括手术、化学疗法、放射疗法、靶向疗法、免疫疗法和激素疗法[5, 6]。尽管化学疗法和放射疗法具有细胞停滞和细胞毒性能力 [7],但这些方法通常与急性副作用和高复发风险有关。最常见的副作用包括神经病、骨髓抑制、胃肠道和皮肤疾病、脱发和疲劳。此外,还有一些药物特异性副作用,如蒽环类药物和博来霉素诱导的心脏毒性和肺毒性[8](图 1)。
<图片>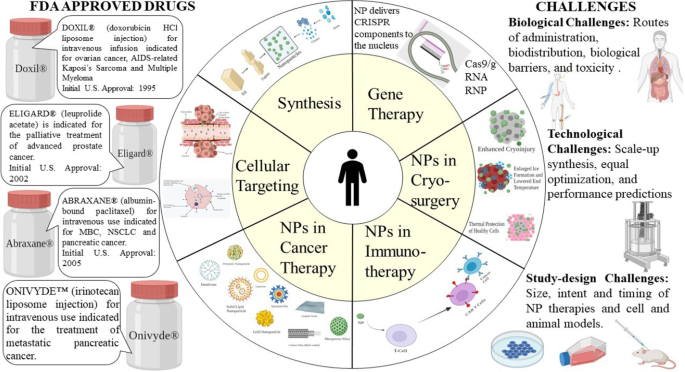
用于癌症治疗的纳米颗粒
图>靶向治疗的出现推动了精准治疗的发展[9]。但仍存在许多不可避免的不良反应,如多重耐药性,限制了治疗效果[8]。免疫治疗药物不仅通过治疗原发性癌症,而且通过预防远处转移和降低复发率,取得了可喜的成果 [10]。然而,自身免疫性疾病是免疫疗法的主要副作用。此外,研究和证据表明免疫疗法对实体瘤的疗效不如淋巴瘤[11]。这些癌症会产生一种不寻常的细胞外基质 (ECM),这对于免疫细胞的浸润非常具有挑战性 [12]。这些新发展的靶向疗法和免疫疗法会干扰对恶性行为和表皮和真皮的正常稳态功能至关重要的信号通路,并导致皮肤病学不良事件 (dAE) [13]。
考虑到所有这些细节,近年来寻求癌症精确治疗的新策略的需求得到了发展。最近已经做出努力来解决使用纳米颗粒的现有治疗方法的局限性。基于纳米颗粒的药物递送系统具有良好的药代动力学、精确靶向、减少副作用和耐药性,在癌症治疗和管理中体现了优势[14, 15]。
随着纳米技术的进步,许多纳米治疗药物已经商业化并广泛上市,自2010年以来,还有更多进入临床阶段。纳米治疗药物在给药系统和抗肿瘤多药领域取得了进展通过为药物联合治疗和抑制耐药机制提供机会 [16]。 1960 年代,苏黎世联邦理工学院率先将纳米技术应用于医学 [17]。事实证明,这种组合是开发各种诊断设备和更好疗法的更好结合。本综述主要围绕纳米药物应用的基本原理、当前挑战前景和未来研究路径进行阐述。
纳米粒子
纳米粒子 (NP) 在技术上被定义为一维小于 100 纳米的粒子,具有独特的特性,通常在相同材料的大块样品中找不到 [18]。根据纳米粒子的整体形状,这些可以分为 0D、1D、2D 或 3D [19]。纳米粒子的基本组成非常复杂,包括表层、壳层和核,核是纳米颗粒的中心部分,通常称为纳米颗粒本身[20]。由于具有高表面积:体积比、相异性、亚微米尺寸和增强的靶向系统等特殊特性,这些材料在多学科领域中获得了很多重要性。
发现 NPs 具有深层组织渗透,以增加增强的渗透性和保留 (EPR) 效果。此外,表面特征通过有效穿过上皮开窗影响生物利用度和半衰期 [21]。例如,涂有聚乙二醇 (PEG)(一种亲水性聚合物)的 NP 会降低调理作用并避免免疫系统清除 [22]。此外,可以通过操纵颗粒聚合物特性来优化药物或活性部分的释放速率。总之,NPs的独特特性调节了它们在癌症管理和治疗中的治疗效果。
NPs 的合成
NP 具有不同的形状、大小和结构。为了实现这一点,采用了多种合成方法。这些方法可以大致分为两大类:1) 自底向上的方法和 2) 自顶向下的方法。这些方法可以根据反应条件和操作进一步分为不同的子类(图 2)。
<图片>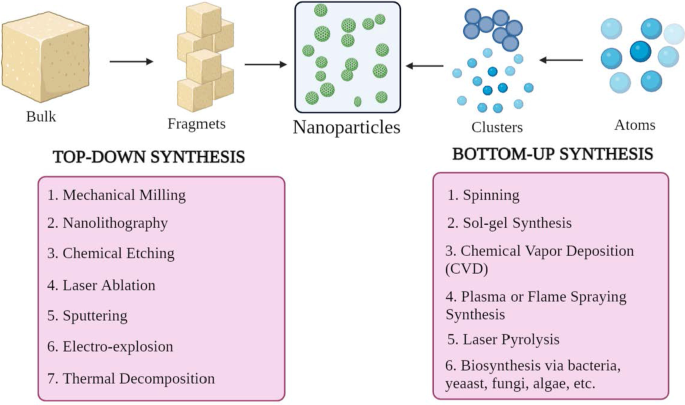
NP合成的分类a 自上而下和 b 自下而上的方法
图>自下而上的方法
这种方法涉及从原子到簇再到 NP 的构建材料,即从更简单的物质构建,因此称为构造方法 [23]。一些常用的方法有纺丝、溶胶凝胶合成、化学气相沉积(CVD)、等离子体或火焰喷涂合成、激光热解和生物合成。
自上而下的方法
它也被称为破坏性方法,它减少大块材料或物质以合成 NPs。一个较大的分子被分解或分解成较小的单元,这些单元被转化为 NPs [24]。它包括机械铣削、纳米光刻、化学蚀刻、激光烧蚀、溅射、电爆炸和热分解等技术。
值得注意的是,纳米颗粒的大小、形状和电荷等形态参数可以通过改变反应条件和其他合成参数来改变 [25]。此外,生长机制也决定了纳米颗粒的化学性质。因此,了解生长机制对于合成所需的纳米颗粒至关重要。
细胞定位机制
对于有效的癌症治疗,必须开发或设计一种药物或基因传递系统,该系统具有出色的靶向肿瘤细胞的能力,而不会影响正常的健康细胞。它增强了治疗效果,从而保护正常细胞免受细胞毒性的影响。它可以通过将 NPs 有组织地输送到肿瘤微环境 (TME) 中来实现,间接靶向癌细胞。这些纳米制剂应该通过许多生理和生物屏障。这些屏障是由多层(上皮、内皮和细胞膜)和成分(机械和物理化学屏障以及酶屏障)组成的复杂系统。这些事实对 NPs 的大小、生物相容性和表面化学提出了规范,以防止非特异性靶向。然而,单纯的 NP 药物分子的胞质内化并不意味着它到达其亚细胞靶点。必须进行特定的工程和优化才能实现细胞或核靶向。
到目前为止,已经进行了几项研究,还有几项研究正在进行中,以发现基于 NP 的药物靶向设计。这些纳米载体通常应具有某些基本特征,例如 1)在到达目标 TME 之前在血管系统(血液)中保持稳定的能力,2)逃避网状内皮系统(RES)清除,3)逃避单核吞噬细胞系统( MPS),4) 通过肿瘤血管系统在 TME 中积累,5) 高压渗透到肿瘤液中,以及 6) 到达靶点并仅与肿瘤细胞相互作用 [26]。表面功能化、理化性质和病理生理特征等重要方面调控着纳米粒药物靶向的过程。
通常,被认为适用于癌症治疗的 NPs 的直径范围为 10-100 nm。为了了解 NP 载体与癌细胞和肿瘤生物学之间的相互作用和串扰过程,解决靶向机制很重要。靶向机制大致可分为被动靶向和主动靶向两大类。
被动定位
在 1980 年代后期发现了癌细胞中少数大分子优先积累的观察结果。据报道,第一个在肿瘤中积累的大分子是 Matsuura 和 Maeda [27] 的聚(苯乙烯-共-马来酸)-新制癌素(SMANCS)。在进一步的研究中,这种优先分布归因于在受损肿瘤血管中发现的开窗和淋巴引流不畅,其合并被称为“增强渗透和保留效应”。
在缺氧或炎症等某些条件下,血管的内皮层变得更具渗透性 [28]。在缺氧情况下,快速生长的肿瘤细胞往往会启动更多的血管或吞噬现有的血管来应对。这个过程被称为新血管形成。这些新血管是渗漏的,因为它们有大孔,与正常血管相比,导致肿瘤血管的渗透选择性较差 [29, 30]。这些大孔或开窗的范围从 200 到 2000 nm,具体取决于癌症类型、TME 和定位 [31]。这种快速且有缺陷的血管生成对外渗几乎没有抵抗力,并允许纳米粒从这些血管中扩散并最终聚集在癌细胞内。
在正常组织中,ECF(细胞外液)流入淋巴管的过程经常以 0.1–2 µm/s 的平均流速发生,从而保持持续的引流和更新 [32]。当肿瘤形成时,淋巴功能会脱轨,导致组织间液摄取量减少 [33]。这一特征有助于 NPs 保留,因为它们没有被清除并囤积在肿瘤间质中。这个过程表示 EPR 效果的增强保留部分。这种特殊功能不适用于循环时间短的分子,并且会迅速从癌细胞中清除。因此,为了改善这种情况,通常将这些小分子封装在纳米药物载体中以增强其药代动力学,提供肿瘤选择性并减少副作用[34]。
在 EPR 效应上,TME 是被动瞄准的一个重要特征。快速增殖的肿瘤细胞的重要代谢特征之一是糖酵解。它是细胞分裂的主要能量来源 [35],并使周围环境呈酸性。可以利用 TME 降低的 pH 值来使用在低 pH 值下释放药物的 pH 敏感纳米颗粒 [36]。
这种类型的肿瘤靶向被称为“被动”。被动靶向主要依赖于不同的肿瘤生物学(血管分布、渗漏性)和载体特性(大小和循环时间)。这种类型的肿瘤靶向不具有针对某些类型肿瘤细胞的特定配体。 EPR 效应在很大程度上依赖于基本的肿瘤生物学,例如 1) 血管生成和淋巴管生成的程度或程度,2) 血管周围肿瘤侵袭的程度或程度,以及 3) 肿瘤内压。这些因素结合纳米颗粒的理化特性,决定了纳米颗粒给药系统的效率(图 3)。
<图片>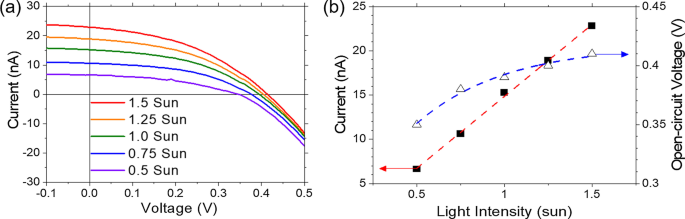
被动细胞靶向
图>被动定位示例
紫杉烷是用于癌症治疗的最成功的药物组之一。紫杉醇已显示出对多种癌症的强大功效。乳腺癌、肺癌(小细胞和非小细胞)和卵巢癌是紫杉类治疗最多的组织学类型。 2005 年,美国 FDA 批准了 Abraxane®(白蛋白结合紫杉醇,Abraxis Bio-Sciences),用于治疗晚期或转移性乳腺癌(MBC)。
Abraxane® 是一种抗微管药物,通过防止解聚来稳定微管。它发生在药物促进微管蛋白二聚体组装微管时。这种获得的稳定性阻碍了微管重组,这在间期和有丝分裂细胞功能中非常重要。在细胞周期和有丝分裂期间,紫杉醇(一种常用的紫杉烷)分别触发不寻常的微管阵列和多个紫杉。在胰腺癌异种移植小鼠模型中,Abraxane® 单独或与另一种细胞毒剂(如吉西他滨)联合减少胰腺基质 [37]。
Genexol PM® 是一种创新的紫杉醇纳米制剂和不含 CrEL 的无菌冻干聚合物胶束制剂。根据试验,发现 Genexol PM® 在裸鼠中的最大耐受剂量 (MTD) 高出三倍。此外,生物分布在肝、脾、肾和肺等不同组织中的水平高出两到三倍,在癌细胞中更为突出。它已在韩国获批用于治疗 MBC。在美国治疗胰腺癌仍在II期临床研究中[38]。
DaunoXome®(脂质体柔红霉素;Gilead Science/Diatos)是一种抗癌药物,可减少肿瘤细胞的生长。活性物质是柔红霉素。它是一种独特的柔红霉素(脂质体形式)配方,用于治疗卡波西肉瘤,这是一种影响皮肤、肺和肠道的癌症。 1996 年美国 FDA 批准[39]。
虽然新生血管形成和血管生成影响 NP 扩散,但它会导致更大的间质压力,从而抑制 NPs 的积累。而且,由于血供异质,肿瘤细胞的生长是不规则的,即靠近血管的细胞比远离血管的细胞分裂得更快,或者在血管内形成核心的缺氧或坏死区深处的细胞分裂得更快。肿瘤。这种不规则的渗漏会导致高间质压力,阻碍药物输送和积累,并减缓新血管形成过程 [34]。然而,可以通过机械或化学方式控制 EPR 效果。这些包括一氧化氮、过氧硝酸盐、缓激肽、VPF(血管通透性因子)、超声、放射、热疗等,但存在一定的局限性和禁忌症。
主动定位
主动靶向取决于特定的配体或分子,如转铁蛋白和叶酸,它们与在靶细胞(患病器官、组织、细胞或亚细胞结构域)上特异性表达或过表达的分子或受体结合[40]。这种类型的靶向称为配体介导的靶向 [41]。在这里,拥有具有特定功能(例如保留和摄取)的配体的 NP 需要位于目标附近,以便有更大的亲和力。该策略增强了纳米颗粒与癌细胞结合的变化,增强了药物渗透。 1980 年观察到的最重要的迹象是在脂质体表面接枝抗体 [34],然后是其他各种类型的配体,如肽、适体。因此,主要方法旨在在不波动总生物分布的情况下增加 NP 与目标之间的串扰 [42]。主动靶向或配体介导的靶向的重要机制是通过靶底物受体识别配体。示例性配体可以包括蛋白质、肽、抗体、核酸、糖、小分子如维生素等[43]。最常研究的受体是转铁蛋白受体、叶酸受体、糖蛋白和表皮生长因子受体 (EGFR)。配体-靶标相互作用通过受体介导的内吞作用触发膜的折叠和 NP 的内化。主动靶向发生的机制有多种。大多数肿瘤靶向通常是通过 NPs 靶向肿瘤细胞完成的。这个过程提高了细胞渗透。如前所述,转铁蛋白是被广泛研究的受体之一。它是一种血清糖蛋白,有助于将铁转运到细胞中。发现这些受体在大多数肿瘤细胞中过度表达,尤其是实体瘤,而在健康细胞中表达水平较低。因此,我们可以用专门针对转铁蛋白的相关配体修饰 NP [44]。例如,A2780 卵巢癌细胞过度表达转铁蛋白。转铁蛋白修饰的 PEG-磷脂酰乙醇胺 (Tf-Mpeg-pe) NPs 使用此功能,专门针对此类细胞 [45]。另一种替代方法是靶向与癌细胞相邻的细胞,例如血管生成内皮细胞。这些细胞还与肿瘤血管密切接触。这种策略可以通过减少癌细胞的血液供应来造成缺氧和坏死。已经发现肿瘤组织比正常组织酸性更强。 Warburg 效应对此进行了广泛的解释 [46]。这解释了癌细胞代谢转变为糖酵解,形成乳酸。当乳酸积累时,细胞就会死亡。为了应对这种情况,细胞开始过度表达质子泵,将多余的乳酸泵入细胞外环境,使其酸性更强。因此,对基于脂质体的pH敏感给药系统进行了研究。
NPs 的多价性质改善了配体包被的 NPs 与靶癌细胞的串扰。此类 NP 的设计很复杂,因为 NP 结构和配体-靶标化学会影响整个方法的功效。其他因素,如给药途径、理化特性(如配体密度 [47] 和纳米颗粒的大小 [8])也有助于该系统的成功(图 4)。
<图片>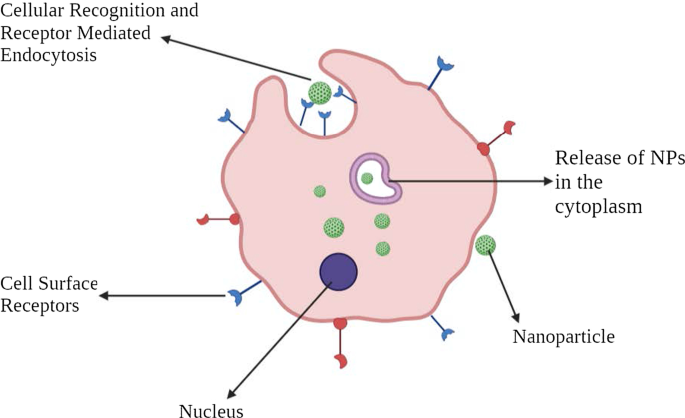
主动细胞靶向的图示
图>主动定位示例
EGFR 是酪氨酸激酶 (TK) 受体 ErbB 家族的成员,在各种类型的癌症中过度表达,尤其是鳞状细胞组织学。具有抗EGFR-PEG-AuNPs和抗IgG-PEG-Au纳米颗粒的金纳米颗粒可用于靶向人SCC[48]。
Herceptin® 是一种治疗药物,靶向在乳腺癌细胞表面过度表达的人类 EGF 受体 2 (HER2)。开发了靶向 HER2 的聚乙二醇化脂质体阿霉素以降低心脏毒性,这是蒽环类药物的已知副作用 [49]。
肿瘤内皮表面表达一种称为血管细胞粘附分子-1 (VCAM-1) 的糖蛋白,其参与血管生成过程。一项研究强调了在乳腺癌模型中靶向 VCAM-1 的 NP,表明其潜在作用 [50]。
叶酸,也称为维生素 B9,在核苷酸合成中至关重要。叶酸被细胞上表达的叶酸受体内化。然而,肿瘤细胞过度表达 FR-α(叶酸受体的 α 异构体),而 FR-β 在液体癌细胞中过度表达 [51]。 NPs靶向叶酸受体目前已用于特定的癌症治疗[52, 53]。
癌症治疗中的纳米粒子
广泛用于给药系统的纳米颗粒包括有机纳米颗粒、无机纳米颗粒和杂化纳米颗粒(图5)。
<图片>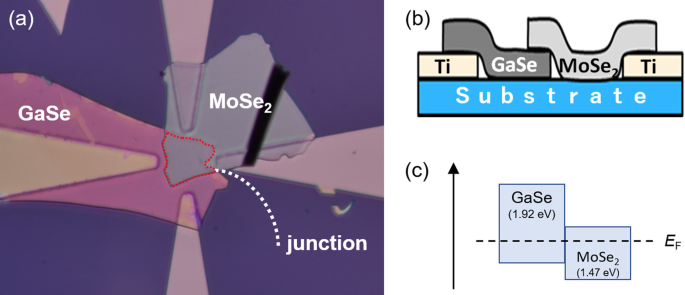
用于癌症治疗的各类纳米材料
图>有机纳米粒子
聚合物纳米粒子
聚合物纳米粒子 (PNP) 被明确定义为“胶体大分子”,具有由不同单体形成的特定结构结构 [54]。药物被包裹或附着在 NPs 的外部,形成纳米球或纳米胶囊,以在靶标中实现受调控的药物释放 [55]。最初,PNP 由不可生物降解的聚合物组成,例如聚丙烯酰胺、聚甲基丙烯酸甲酯 (PMMA) 和聚苯乙烯 [56]。然而,由于难以从系统中消除这些物质,这些物质的积累会导致毒性。可生物降解的聚合物,例如聚乳酸、聚(氨基酸)、壳聚糖、藻酸盐和白蛋白,现在正在使用中,并且已知可以降低毒性并增强药物释放和生物相容性 [57]。经证实的研究表明,通过用聚山梨酯涂覆 PNP 和使用聚山梨酯表面活性剂效果。外涂层增强了纳米颗粒与血脑屏障 (BBB) 内皮细胞膜的相互作用 [58]。
一项研究表明,在大鼠异种移植神经胶质瘤模型中,装有吲哚美辛的纳米胶囊可显着减小肿瘤大小并提高存活率 [59]。这是一个不断发展的领域,有十多种含有抗癌药物的聚合物 NP 正在临床开发中。一些例子包括紫杉醇 poliglumex (Xyotax)、PEG-喜树碱 (Prothecan)、改性葡聚糖-喜树碱 (DE 310)、HPMA 共聚物-DACH-铂酸盐 (AP5346)、HPMA 共聚物-铂酸盐 (AP 5280)、HPMA 共聚物-紫杉醇 ( PNU166945) 和 HPMA 共聚物-多柔比星半乳糖胺 (PK2) [60]。
树枝状聚合物
树枝状聚合物是具有明确超支化结构的球形聚合物大分子。高度支化的结构是树枝状聚合物的特征。通常,树枝状聚合物的合成是通过使氨核与丙烯酸反应而引发的。该反应导致形成“三酸”分子,该分子进一步与乙二胺反应生成“三胺”,即 GO 产物。该产物进一步与丙烯酸反应生成六酸,进而生成“六胺”(第一代)产物,依此类推[61]。通常,树枝状聚合物的大小范围为 1-10 nm。然而,尺寸可能达到 15 nm [62]。鉴于它们的特定结构,如定义的分子量、可调整的分支、生物利用度和电荷,它们被用于靶向核酸。一些广泛使用的树枝状大分子有聚酰胺胺(PAMAM)、PEG(聚(乙二醇))、PPI(聚丙烯亚胺)和TEA(三乙醇胺)[63]。
PAMAM 树枝状聚合物最初旨在实现 MDR 管理。 DNA组装的PAMAM树枝状聚合物已被广泛描述。与接受单药化疗的动物相比,合成的树枝状大分子显着延迟了上皮癌异种移植物的生长[64]。
mAb 纳米颗粒
单克隆抗体因其特殊的靶向能力而被广泛用于癌症治疗 [65]。这些 mAb 现在与 NP 结合形成抗体-药物偶联物 (ADC)。这些被证明比单独的细胞毒性药物或 mAb 具有高度的特异性和吸引力。例如,在 HER2 阳性乳腺上皮细胞对照中,由紫杉醇核心和曲妥珠单抗修饰的表面组成的抗体药物 NP 比单药紫杉醇或曲妥珠单抗具有更好的抗肿瘤功效和更低的毒性[66]。
细胞外囊泡
细胞外囊泡 (EV) 是双层磷脂囊泡,大小范围为 50-1000 nm [67]。 EV 由不同的细胞类型持续分泌,并且在来源、大小和组成上各不相同。 EV 分为三类:1) 外泌体,2) 微泡,和 3) 凋亡小体 [68]。与外泌体结合的 NPs 被广泛使用,因为它们具有与原始细胞非常相似的脂质和分子。此外,它们逃避免疫监视并在癌细胞内迅速内化。它们充当天然载体,将细胞毒性药物和其他抗肿瘤药物输送到目标部位。装载多柔比星 (exoDOX) 的外泌体是最好的例子。 exoDOX 用于治疗乳腺癌,与多柔比星的保守治疗相比,通过增强细胞毒性和避免心脏毒性,显示出很好的效果 [69]。与合成 NPs 相比,外泌体 NPs 具有内在的生物相容性特征、先进的化学稳定性和细胞内通讯。然而,缺乏外泌体分离和纯化标准条件等缺点是至关重要的,需要解决[70, 71]。
脂质体
这些是包含磷脂的球形囊泡,磷脂可以是单层或多层的,以包裹药物分子 [72]。脂质体具有独特的特性,例如低内在毒性、弱免疫原性和生物惰性 [73]。脂质体是 1965 年批准的第一种纳米级药物 [74]。典型的脂质体结构由“亲水核”和“疏水磷脂双层”组成。这种独特的结构使它们能够同时捕获亲水性和疏水性药物,从而有效地保护被捕获的药物在循环中免受环境降解 [75]。
脂质体通过展示更高的抗肿瘤功效和增强的生物利用度,为阿霉素、紫杉醇和核酸等药物递送提供了极好的平台 [76]。 Doxil® 和 Myocet® 是经批准的基于脂质体的柔红霉素制剂,用于治疗 MBC [77, 78]。然而,由于脂质体纳米粒的包封率降低、MP去除速度快、细胞吸附性强、保质期短等缺点,限制了脂质体纳米粒的应用。
固体脂质纳米颗粒 (SLN)
它们是由磷脂单层、乳化剂和水组成的胶体纳米载体(1-100 nm)[79]。这些被称为零维纳米材料。脂质成分可以是甘油三酯、脂肪酸、蜡、类固醇和聚乙二醇化脂质 [80]。 Unlike conventional liposomes, SLNs have a “micelle-like structure” within which the drug is entrapped in a non-aqueous core. Examples include mitoxantrone-loaded SLN, which has shown reduced toxicity and enhanced bioavailability [81]. The incorporation of doxorubicin and idarubicin by SLN in “P388/ADR leukemia cells” and the “murine leukemia mouse model” has shown positive results [82].
Nanoemulsions
Nanoemulsions are colloidal NPs with heterogeneous mixtures of an oil droplet in aqueous media ranging from 10–1000 nm [83]. Three representative types of nanoemulsions can be made in:1) oil-in-water system, 2) water-in-oil system, and 3) bi-continuous nanoemulsions. Membrane-modified nanoemulsions have been extensively studied. For instance, nanoemulsions loaded with spirulina and paclitaxel showed an improved anti-tumor effect by regulating immunity through TLR4/NF-kB signaling pathways [84]. Nanoemulsion consisting of rapamycin, bevacizumab, and temozolomide is known to treat advanced melanoma [85]. Nanoemulsions are different from liposomes and certainly have enhanced characteristics than others, such as optical clarity, stability, and biodegradability [86]. However, there are challenges to clinical applications of these nanoemulsions as these involve high temperature and pressure and instruments such as homogenizers and microfluidizers that are expensive.
Cyclodextrin Nanosponges
Cyclodextrins are usually used as stabilizers to increase the drug loading capacity of NPs [87]. Nanosponges are tiny, mesh-like structures [88]. Β-cyclodextrin nanosponges loaded with paclitaxel have shown sound cytotoxic effects in MCF-7 cell line culture [89]. Similarly, camptothecin has shown improved solubility and stability when formulated with cyclodextrin-based nanosponges [90].
Inorganic Nanoparticles
Carbon Nanoparticles
Carbon NPs as the name suggests are based on the element carbon. They have been widely utilized in medical arenas because of their optical, mechanical, and electronic properties combined with biocompatibility [91]. Due to their inherent hydrophobic nature, carbon NPs can encapsulate drugs through π-π stacking [92]. Carbon NPs are further categorized into graphene, carbon nanotubes, fullerenes, carbon nanohorns, and graphyne. Although all these are carbon-based, they vary in their structure, morphology, and properties.
“Graphene” is 2D crystal with sp2-hybridized carbon sheet that holds extraordinary mechanical, electrochemical, and high drug loading properties. Further, based on composition, properties, and composition, graphene can be divided as follows:1) single-layer graphene, 2) graphene oxide (GO), 3) reduced graphene oxide (rGO), and 4) multi-layer graphene [93]. GO and rGOs are widely used due to their ability to target hypoxia [94] and irregular angiogenesis in TME [95]. Studies have shown that GO-doxorubicin exhibits higher anticancer activities in cellular models of breast cancer [96].
Fullerenes are large carbon-cage molecules composed of carbon allotrope with different conformation types such as sphere, ellipsoid, or tube. They are the most widely studied nanocarriers as they have typical structural, physical, chemical, and electrical properties [97]. These are used in photodynamic therapy as they have triple yield and generate oxygen species due to the presence of extended π-conjugation and the ability to absorb light [98]. PEG-modified fullerenes showed promising photodynamic effects on tumor cells [99].
Carbon nanotubes (CNTs) are cylindrical tubes, most often considered as rolls of graphene, were discovered in the late 1980s. They are classified into two groups:1) single-walled CNTs and 2) multi-walled CNTs. As they are carbon-based, they can bring upon immune response by interacting with immune cells, thereby suppressing the tumor growth. Traditionally, they have been used as DNA delivery vectors and for thermal ablation therapy. For instance, a fluorescent single-walled CNT with mAb encapsulating doxorubicin is used to target colon cancer cells. Such CNTs form a complex which is effectively engulfed by the cancer cells leading to the intracellular release of doxorubicin, whereas the CNTs are retained in the cytoplasm [100].
Quantum Dots
Quantum dots are typically nanometer-scale semiconductors with a broad spectrum of absorption, narrow emission bands, and high photostability, allowing them to be widely used in biological imaging [101]. Based on carbon, these are divided into:1) graphene quantum dots, 2) nanodiamond quantum dots, and 3) carbon quantum dots. Besides biological imaging, quantum dots are being actively investigated in cancer treatment. The most commonly used quantum dots is graphene quantum dots due to their inherent biocompatibility and rapid excretion. For example, quantum dots aptamer—doxorubicin conjugate targets prostate cancer cells [102]. However, the deficiency of optimized process in producing quantum dots is the major obstacle.
Metallic Nanoparticles
Metallic nanoparticles are commonly explored in “biological imaging” and targeted DDS due to their remarkable optical, magnetic, and photothermal properties. Some of the most commonly used metallic NPs are gold NPs, silver NPs, iron-based NPs, and copper NPs. Gold NPs are used as intracellular targeting drug carriers because the size and surface properties are easily controlled [103]. Moreover, their visible light extinction behavior makes it possible to track NP trajectories in the cells. “Anti-HER2 functionalized gold-on-silica nanoshells” have been shown to aim HER2 positive breast cancer cells [104]. Combidex®, an iron oxide NP formulation, is presently in the late-stage clinical testing phase to detect nodal metastases [105]. Feraheme®, a ferumoxytol containing iron oxide NP formulation, is used to treat iron-deficiency anemia. This is also used to treat nodal metastases in prostate and testicular cancer and was approved by FDA in June 2009 [106, 107].
Magnetic Nanoparticles
Magnetic NPs are generally used in MRI imaging, and drug delivery contains metal or metal oxides. These are usually covered with organic substances like polymers and fatty acids to enhance stability and biocompatibility [108]. LHRH-conjugated superparamagnetic iron oxide NPs are effective in targeting and imaging of breast cancer [109]. Moreover, magnetic NPs are used in magnetic hyperthermia for thermal ablation of cancer cells [110, 111]. Some of the magnetic NPs that are in the market or in the clinical trial phase are Feridex® and Resovist® for liver metastasis and colon cancer [112].
Calcium Phosphate Nanoparticles
“Calcium phosphate NPs” is biologically compatible, biodegradable, and do not cause any harsh adverse reactions. Hence, they are used as a delivery agent for insulin, growth factors, antibiotics, and contraceptives [113]. They are also used in the delivery of oligonucleotides and plasmid DNA [114]. Calcium phosphate NPs combined with either viral or non-viral vector has been positively used as delivery vectors in cellular gene transfer. A “liposomal nanolipoplex formulation” of calcium and glycerol has shown decreased toxicity and enhanced transfection features [115, 116].
Silica Nanoparticles
Silica being a significant component of many natural materials was only studied concerning biology recently. Silica NPs are commonly used to deliver genes by functionalizing the NP surface with amino-silicanes [117]. N-(6–aminohexyl)–3–aminopropyl–trimethoxysilane functionalized silica NPs have shown excellent efficiency in the transfection of Cos-1 cells with minimal toxicity and is now commercially available [118]. Mesoporous silica NPs are considered one of the best drug carriers due to their better pharmacokinetic properties. They have been extensively used in immunotherapy. According to a study, colorectal cancer cells have shown successful uptake of camptothecin-loaded mesoporous silica NPs.
Mechanism of NPs in Overcoming Drug Resistance
Drug resistance is one of the chief problems in cancer therapy and management. It prevails across all types of cancer and all possible treatment modalities. Drug resistance is a phenomenon that results when diseases become tolerant to pharmaceutical treatments. Drug resistance can be classified into two types:1) innate and 2) acquired [119]. Innate resistance usually results from pre-existing mutations in the genes that are involved in cell growth or apoptosis. Acquired resistance is defined as the type of resistance that is developed after a particular anti-tumor treatment, which may result from the development of new mutations or from alterations in the TME during treatment. Nanoparticles, due to their extraordinary ability to co-encapsulate multiple therapeutic agents, can also be used to overcome cancer-related drug resistance.
Targeting Efflux Transporters
Efflux transporters are classified under the family of “ATP-binding cassette (ABC) transporters.” These have a significant role in MDR. The primary function of these transporters is to pump out drugs out of the cell and reduce the concentration. “P-glycoprotein (P-gp)” is one such efflux transporter that is overexpressed by drug-resistant cancer cells [120, 121].
Overexpression of P-gp has been linked with inadequate treatment response, especially in breast cancer [122] and ovarian cancer [123]. NPs can be used to tackle efflux pumps. As NPs internalize the cell via “endocytosis” instead of diffusion and release the drug at the “perinuclear site,” which is distant from active efflux pumps, NPs can bypass the efflux pumps [124]. Besides, by modifying the control of drug releases, such as by utilizing low pH levels and redox as triggers, NPs can effectively bypass efflux pumps [125, 126].
Combination therapy is yet another method to overcome MDR. NPs can be loaded with multiple drugs within a single drug carrier [127]. Inhibiting efflux transporter expression instead of just dodging them would be another viable option. This can be achieved by building NPs in such a way that it can entrap both efflux pump inhibitors and chemotherapy agents [128]. A recent study positively reflected upon reversing MDR in breast cancer cells by using NPs that co-deliver COX-2 inhibitors and doxorubicin [129]. Similarly, using silica NP that encapsulates miRNA-495 and doxorubicin has proved effective in overcoming drug resistance in lung cancer cells [130]. Another interesting study found out that using NPs in the tumor neo-vasculature targeting KDR receptors is a more effective anti-tumor function than P-gp inhibitor combination therapy. Yet, another way of overcoming drug resistance is by depleting the source of ATP, which is essential for the functioning of ABC transporters. This can be done by targeting mitochondria which leads to a decrease in ATP production.
Targeting an Apoptotic Pathway
Cancer cells proliferate due to faulty apoptotic machinery and upsurge their survival adding to drug resistance [131]. The faulty apoptotic pathway gets activated by “deregulation of Bcl-2” and “nuclear factor kappa B (NF-κB).” These are the most widely investigated anti-apoptotic proteins and can be potentially used as the target for reversing drug resistance. Using a classic process of co-delivery of “Bcl-2 siRNA and chemotherapeutics” by NPs is a way to overcome MDR [132]. NF-κB inhibitors have been used in combination with “pyrrolidine dithiocarbamate (PDTC)” [133] and curcumin [134]. Besides suppressing anti-apoptotic factors, triggering pro-apoptotic factors is another to fight “apoptotic pathway-mediated drug resistance.” For instance, a combination of ceramide and paclitaxel is a good example [135]. Ceramide restores the expression of a chief tumor suppressor, p53 protein, by regulating alternative pre-mRNA splicing. Delivering ceramide via NPs is an excellent way to correct the p53 missense mutation [136]. Owing to its potential, a combination of ceramide and paclitaxel has shown significant therapeutic efficacy in cancer drug resistance models. Transfecting the p53 gene by cationic SLNs has been reported in lung cancer cases [137]. Similarly, transfecting the p53 gene by PLGA has been carried out in breast cancer cells models that have shown potent induction of apoptosis and inhibition of tumor growth [138].
Some NP-based DDS act by impeding efflux pumps and encouraging apoptosis [139]. A pioneering study conducted to prove both pump- and non-pump-mediated drug resistance used an “amphiphilic cationic NP” entrapping paclitaxel and Bcl-2 converter gene in drug-resistant liver cancer models. NP complex diminished P-gp-induced drug efflux and the apoptosis activation. Similarly, co-delivery of “doxorubicin and resveratrol encapsulated in NPs” has shown noteworthy cellular toxicity on doxorubicin resistance breast cancer cells by downregulating the expression of Bcl-2 and NF-κB, thereby initiating apoptosis as well as through the inhibition of efflux transporter expression [140]. A similar study was done on multi-drug resistant prostate cancer cells by using folic acid-conjugated planetary ball milled NPs encapsulated with resveratrol and docetaxel. This worked by downregulating anti-apoptotic gene expression while inhibiting ABC transporter markers [141].
Targeting Hypoxia
Hypoxia is yet an additional aspect that backs MDR [142]. Due to abnormal blood vessels in the vicinity of the tumor and due to the increasing demand of oxygen by the rapidly growing tumor, some tumor cells are repeatedly in a hypoxic condition. The part of the tumor that is in hypoxic condition often escapes from the chemotherapy drugs. Hypoxia creates an oxygen ramp inside the tumor that intensifies tumor heterogeneity, encouraging a more aggressive phenotype. Moreover, the hypoxia condition has been established to facilitate the overexpression of efflux proteins [143]. The major protein, “hypoxia-inducible factor 1α (HIF-1α)” acts an important role. Hence targeting HIF-1α or silencing HIF-1α gene is a way to overcome drug resistance. NPs containing HIF-1α siRNA can be used to reduce hypoxia-mediated drug resistance [144]. Instead of directly targeting HIF-1α, indirect inhibition of HIF-1α signaling can be used. For example, the “PI3K/Akt/mTOR pathway” is known to control the expression of HIF-1α. Inhibition of this pathway effectively downregulates the expression of HIF-1α, which enhances the sensitivity of MDR cells to cancer treatment [145]. NPs like PLGA-PEG and PEGylated and non-PEGylated liposomes can be used effectively. In addition, “heat shock protein 90 (HSP90)” is needed for transcriptional activity of HIF-1 and inhibition of HSP90, which downregulates the expression of HIF-1α [146]. The HSP90 inhibitor in “17AAG loaded NPs” has dramatically improved MDR in bladder cancer treatment [147].
Nanoparticles and Proteomics
When NPs are subjected to the biological system, they are surrounded by cellular and serum proteins which form a structure known as protein corona (PC) [148]. Based on the degree of interaction of these proteins with the NPs, there are classified into the hard corona and soft corona. “Hard corona” is formed when these proteins have a high binding affinity towards the NPs. “Soft corona” is produced when these proteins are loosely bound to the NPS. It has been established that the most protein forming a PC first will be eventually substituted by proteins with higher affinities. This is known as Vroman effect [149]. Hence developing the technology that can manufacture NPs with desired properties is essential. Several proteomic approaches such as MS, LC–MS, SDS-PAGE, isothermal microcalorimetry (ITC), etc. [150], are being used. PC affects the crosstalk of NP with the biological setting and thereby governs the application and usage of the same in the medical field.
Cancer proteomics studies the number of proteins in cancer cells and serum, which supports hunting proteins and biomarkers that aids in diagnosis, treatment, and prognosis [151]. It also helps in understanding cancer pathogenesis and drug resistance mechanism. Post-translational modifications (PTMs) play an indispensable part in occurrence, recurrence, and metastasis. Besides using chemotherapy and kinase inhibitors, novel agents like siRNA, mRNA, and gene editing are central therapeutics used with NPs.
Nanotechnology for Small Interfering RNA (siRNA) Delivery
siRNAs are small ds RNA molecules (around 21 nucleotides long) that suppress the expression of genes in the target. This process is known as “RNA interference.” A few siRNA-based NPs that are currently under clinical investigations are ALN-TTR01 that is used to target the transthyretin gene to treat transthyretin-mediated amyloidosis, and Atu027, which is a liposomal siRNA that targets protein kinase N3 and TKM-ApoB that knock downs the expression of ApoB [152, 153].
Nanotechnology for Tumor microRNA Profiling and Delivery
MicroRNAs are a class of endogenous “single-stranded non-coding RNA” molecules that control post-transcription gene expression by blocking translation of the target mRNA or repressing protein production by destabilizing mRNA [154]. These are emerging as vital biomarkers that are a significant target for cancer diagnosis, therapy, and treatment. The base priming nature of nucleic acid forms the very foundation for nanotechnology used miRNA profiling techniques. Several profiling techniques use biosensors or surface plasmon resonance imaging techniques in combination with molecular biology enzymatic reactions. Nanotechnology can be used for the delivery of MicroRNAs. For example, biodegradable polycationic prodrugs showed promising results in the regulation of polyamine metabolism [155]. MicroRNA-loaded polycation-hyaluronic acid NPs of single-chain antibody fragments have shown progressive downregulation of “survivin expression” in high metastatic cancer load in the lung of murine B16F10 melanoma.
DNA Nanotechnology for Cancer Therapy
DNA-based nanostructures have been synthesized for DNA sensors to detect nucleic acid, DNA-coated gold NPs for lead sensing by hybridizing Pb-activated DNAzyme to the linking DNA, scaffolds to organize organics, inorganic, and biomolecules into distinct morphology molecular transporters, and drug delivery (Table 1).
图>Advantages of Nanoparticles in Cancer Therapy
The utilization of nanotechnology in the diagnosis, treatment, and management of cancer has led to a whole new era. NPs, either by active or passive targeting, augment the intracellular concentration of drugs while avoiding toxicity in the healthy tissue. The targeted NPs can be designed and altered as either pH-sensitive or temperature-sensitive to establish and regulate the drug release. The pH-sensitive drug delivery system can deliver drugs within the acidic TME. Similarly, the temperature-sensitive NPs release the drugs in the target site due to changes in temperature brought in by sources like magnetic fields and ultrasound waves. In addition, the “physicochemical characteristics” of NPs, such as shape, size, molecular mass, and surface chemistry, have a significant part in the targeted drug delivery system. Further, NPs can be modified according to the target and used to target a particular moiety.
Conventional chemotherapy and radiation therapy have several disadvantages concerning efficacy and side effects because of uneven dispersal and cytotoxicity. Therefore, cautious dosing is required that effectively kills cancer cells without any significant toxicity. To reach the target site, the drug has to pass several fortifications. Drug metabolism is a very complex process. In physiological conditions, the drug needs to pass TME, RES, BBB, and kidney infiltration. RES or macrophage system is made up of “blood monocytes, macrophages, and other immune cells” [160]. MPS in the liver, spleen, or lungs react with the drugs and activate “macrophages or leukocytes” that rapidly remove the drug. This leads to a short half-life of the drug [161]. To overcome this, NPs with “surface modification,” such as PEG, bypass this mechanism and increase the “drug half-life.” Besides, kidney infiltration is a crucial function in the human body. Proper kidney infiltration thus minimizes the toxicity caused by NPs.
The brain-blood barrier (BBB) is a specialized protection structure offered to protect the CNS from harmful and toxic agents. “Brain capillary endothelial cells” are arranged in the form of a wall that provides essential nutrients to the brain. Since the primary function of BBB is to block toxic agents to reach the brain, currently available chemotherapy agents for brain cancer are highly limited to intraventricular or intracerebral infusions [162]. However, NPs are known to cross BBB. Now, several approaches such as EPR effect, focused ultrasound, peptide-modified endocytosis, and transcytosis are used to deliver NPs. Glutathione PEGylated liposome encapsulated with methotrexate showed improved methotrexate uptake in rats [163]. Au-NPs are often used as they have proven to help transport drugs to induce apoptosis [164].
NPs being carriers also increase the drug stability by preventing the degradation of the encapsulated cargo. Additionally, a large volume of drugs can be encapsulated without any chemical reaction. Dry solid dosage forms are more stable than nanoliquid products [165]. Stabilizers can be used to enhance stability. Yet another way to increase stability is to use porous NPs.
Tumor has unique pathophysiology features such as extensive angiogenesis, flawed vascular architecture and defective lymphatic drainage. The NPs use these features to target tumor tissue. Due to reduced venous return in tumor tissue and meager lymphatic clearance, NPs are effectively retained. This phenomenon is known as EPR. Similarly, by targeting the adjacent tissues, tumor-targeting can be accomplished [166].
NPs can be administered through several routes like oral, nasal, parenteral, intra-ocular etc. NPs have a high surface-to-volume ratio and intracellular uptake. Studies have reported that NPs are more effective than microparticles as drug carriers [167].
Nanoparticles in Immunotherapy
The immune system sets an important part in the establishment and development of cancer cells. The advancement of immunotherapy has revolutionized cancer therapy. It is found that NPs not only help in target delivery of chemotherapy but can also be used in combination with immunotherapy. There are several approaches in immunotherapy aimed at activating the immune system against cancer cells [168] by “immune checkpoint blockade therapy,” “cancer vaccine therapy,” “chimeric antigen receptor (CAR)-T cell therapy,” and “immune system modulator therapy” [169,170,171]. NP-based immunotherapy includes “nanovaccines,” “aAPCs (artificial antigen-presenting cells),” and “immunosuppressed TME targeting.”
Nanovaccines specialize in delivering “tumor-associated antigens” and “adjuvants” to antigen-presenting cells, such as dendritic cells (DCs) [172]. Moreover, these can also be employed as adjuvants to enhance “APC antigen presentation” and promote DC maturation that leads to the stimulation of cytotoxic T cells that have anti-tumor function [173, 174]. Liposomes, PLGA NPs, gold NPs are found to have the ability to deliver TAAs into DCs in the cytoplasm [175]. Mesoporous silica, the most used inorganic NP, has exhibited an adjuvant role, leading to immune response stimulation [176]. Artificial APCs interact with MHC-antigen complexes directly which binds to T cells. They also bind to co-stimulatory molecules that bind to co-stimulatory receptors leading to T cell activation [177]. Targeting the immunosuppressed TME is yet another method of using NPs in immunotherapies. This is done by targeting essential cell types in TME such as “tumor-associated macrophages (TAMs),” regulatory T cells, and “myeloid-derived suppressor cells (MDSCs).”
Besides, the combination of chemoimmunotherapy has been demonstrated to be a capable approach in cancer therapy. For instance, a study has shown that co-loading Nutlin-3a, which is a chemotherapeutic agent and cytokine GM-CSF, in “spermine-modified acetylated dextran (AcDEX) NPs” improved cytotoxic CD8( +) T cells proliferation and activated an immune response [178].
“Programmed cell death protein 1 (PD-1)” and “programmed cell death ligand 1 (PD-L1)” are some of the essential immune checkpoints [179]. Hence immune checkpoint inhibitors are used to target these using NPs. According to a study, conventional immune checkpoint inhibitors of PD-L1/PD-1 displayed inconsistent responses. To enhance the chances and bonding of immune checkpoint inhibitors and immune checkpoints, multivalent poly (amidoamine) dendrimers were used. Usage of these dendrimers not only showed enhanced PD-L1 blockade but also showed improved drug accumulation at the tumor site [180].
Nanoparticles in Cryosurgery
Cryosurgery is an advanced practice of freeze-destroying cancer tissue. Although this is less invasive and causes intraoperative bleeding and postoperative complications, certain drawbacks like inadequate freezing capacity and damage to adjacent cells need to be addressed [181]. The rise of nanotechnology has enabled the use of NPs in cryosurgery.
The primary working of nanocryosurgery is introducing NPs with particular properties into the cancer cells and causing freezing [182]. During this process, ice is formed within the cells, which causes damage to it. This is an important process and can be carried out effectively using NPs. The thermal conductivity property of NPs can be exploited, which significantly freeze the tumor tissue and cause tumor damage [183]. Besides, they cool down rapidly, and it is feasible to regulate the “growth direction” and “direction of the ice ball” (Fig. 6).
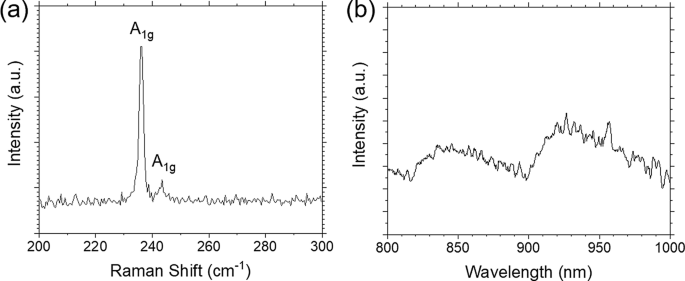
Diagrammatic representation of NPs in cryosurgery
图>When the location of the tumor makes it not feasible for cryosurgery or if other adjacent organs are at risk, there are high chances that the freezing can damage healthy tissue. Recently, phase change materials (PMs) made up of NPs are used to protect the adjacent normal healthy tissue during cryosurgery [184]. For instance, liposome-based microencapsulated phase change NPs have shown incredible results in protecting surrounding healthy tissue [185]. These NPs are deemed to possess large latent heat and low thermal conductivity, making them perfect for cryosurgery.
Significant Challenges in the Clinical Application of Nanoparticles
At present, as nanotechnology has bloomed, the amount of knowledge and research put into nanoparticles has steeply raised. But only a few of them actually make it up to clinical trials. Most of them only halt at in vivo and in vitro stages. Each individual nanoformulation has particular challenges in their clinical translation, but most NPs face similar challenges that can be divided into biological, technological, and study-design related.
Biological challenges include lack of routes of administration, tempering biodistribution, the channel of NPs across the biological barriers, their degradation, and toxicity [186]. NPs are usually injected via intravenous injections directly into the blood, which takes away NPs, making it challenging to stay and interact with the target site. As a result, a high concentration drug is used, which might not provide desired therapeutic effects [187]. However, magnetic NPs can be used to overcome this as many in vivo and in vitro studies have proved the usage of 3D magnetic fields to control the movement of NPs against blood flow. But, the effect of magnetic fields on the human body, crosstalk between magnetic fields, and a large number of NPs has to be researched upon.
Controlling the biological fate of NPs is very hard and needs a lot of focus. Even though NPs are made up of biosafety materials and are modulated accordingly to increase the retention time and half-life, there runs a risk of lung, liver and kidney damage. Some factors that govern toxicity are surface area, particle size and shape, solubility, and agglomeration [188]. NPs have shown greater deposition in the lung with inflammatory, oxidative and cytotoxic effects [189]. Studies reveal that healthy cells often suffer from free radicals generated by NPs [190]. Fabricating NPs with more biocompatible substances like chitosan and materials that disintegrate after near infrared light irradiation may be potential solutions.
Another tricky challenge is avoiding the “mononuclear phagocytic system (MPS).” In biological fluids, NPs adsorb proteins to produce PC, which attacks MPS to uptake NPs. To escape this, NPs have been coated with materials that prevent the formation of the protein corona. However, they have not shown any significant results. Designing NPs that target “macrophages” and using those as new drug vehicles can be pitched to overcome this problem. Currently, preventing macrophage recruitment, depleting and reprograming TAMs, and obstructing “CD47-SIRPα pathways” are commonly used strategies [191].
Technological challenges of NPs include scale-up synthesis, equal optimization, and performance predictions. These are very crucial in safeguarding the clinical success of NPs. Most of the NPs that are used in vivo and in vitro studies are usually produced in minor batches, and scale-up for huge quantities is not constantly feasible given instrumentation and other reasons. The lead clinical candidates that prove to be the best in animal models are not systematically designed optimized. To overcome this, we can use certain methods that can test numerous nanoformulation and by selective iterations selecting a single optimized formulation [192,193,194]. However, such hits shouldn’t be introduced directly in human testing. Predicting nanoparticle efficacy and performance is hard and replicating the in vivo results in human trials is a herculean task. Computational or theoretical modeling along with experimental results can be designed to imitate physiological tissue and surrounding. For instance, organs-on-chips are being actively studied and can improve NP predictions of efficacy and performance.
Study-design challenges like study size, intent, and timing of NP therapies during the therapy impact significantly during clinical studies. Most of the studies revolve around “cell and animal models” that may not provide comprehensible results in human trials. Therefore, the usage of a single model is tough to imitate natural reactions in the human body. In addition, “models of cancer metastasis” should be actively researched as metastasis is one of the significant properties of cancer. Moreover, N = 1 clinical studies will be required if we focus on personalized medicine. This needs to count in many factors such as genetic, environmental, and past medical history. [195, 196]. Another major challenge is that NPs are never used as first-line therapies. Although we have effectively approved nanoformulations, they are usually saved for further treatment if disease progression is found in the clinical trial scenario. Most of the patients have either had progressed on multiple lines of therapies or have gained drug resistance. These situations often skew the clinical trial results and lessen the chance of NP treatment to benefit those who are likely still treatable.
Conclusion and Future Perspective
Nanotechnology has shown a promising new era of cancer treatment by delivering small molecules for cancer detection, diagnosis, and therapy. Cancer therapies based on the exceptional features of NPs are being vastly used in the clinical setting of several cancer types. NP-based DDS is linked with enhanced pharmacokinetics, biocompatibility, tumor targeting, and stability compared to conventional drugs. Moreover, NPs provide an excellent platform for combination therapy which helps in overcoming MDR. With increasing research, several types of NPs, such as polymeric NPs, metallic NPs, and hybrid NPs, have shown improved efficacy of drug delivery. Researchers must be well attentive to the features of the nominated nanoplatforms and the properties of therapeutic agents. However, there are certain limitations like deficiency of in vitro models that precisely replicate in vivo stage, immunotoxicity, the long-term toxicity, and neurotoxicity. Although “nanovaccines” and “artificial APCs” have proved improved efficacy compared to conventional immunotherapy, the clinical efficacy is substandard. The safety and tolerance of these new modalities should to be inspected. Additionally, developing “immunomodulatory factor-loaded NPs” may advance the efficiency of vaccines for immunotherapy.
This is an emerging area, and it is anticipated that with growth in proteomics research on the “mechanism of cancer origin, MDR, occurrence,” more NP-based drugs can be exploited. Compared to the mammoth amount of investigations, only a few NP-based drugs are actually in use, a few others in clinical trials, and most in the exploratory stage. For rational nanotechnology design, more efforts must be reserved in “understanding toxicity, cellular and physiological factors that regulate NP-based drug delivery, EPR, and PC mechanism” in the human body. Based on the evidence cited above, we presuppose that the revolution in clinical translation for NP-based cancer therapy will be attained with nanotechnology and cancer therapy development.
Availability of Data and Materials
Not applicable.
纳米材料
- 5G 的前五个问题和挑战
- 用于灵敏快速检测卵巢癌细胞的柔性石墨烯生物传感器的演示
- 用于改进诊断和治疗应用的多功能金纳米粒子:综述
- 用于合成和生物医学应用的荧光纳米材料的进展和挑战
- 加载白藜芦醇的白蛋白纳米颗粒具有延长血液循环和改善生物相容性的高效靶向胰腺肿瘤治疗
- 用于体内 CT 成像和肾脏清除特性的新型生物相容性 Au Nanostars@PEG 纳米颗粒
- 用于光热疗法和光声成像的聚吡咯涂层铁铂纳米粒子的合成和体外性能
- 5-氨基乙酰丙酸-角鲨烯纳米组件用于肿瘤光检测和治疗:体外研究
- Mg 掺杂对用于增强光催化评估和抗菌分析的 ZnO 纳米颗粒的影响
- 用于提高溶解度的蝌蚪形和球形血红素纳米颗粒的制备和表征
- 用于高效结肠癌基因治疗的基于阳离子胶束的 siRNA 递送
- 了解混合制造的优势和挑战