电化学自组织二氧化钛纳米管阵列综述:合成、修饰和生物医学应用
摘要
通过阳极氧化生长的二氧化钛纳米管以其许多独特和潜在的特性引起了材料科学界的兴趣,并且技术的合成正在走向成熟阶段。本综述将重点关注通过 Ti 金属基板自组织电化学阳极氧化生长的 TiO2 纳米管,重点强调了这种类型的自组织二氧化钛纳米管层的合成以及影响尺寸、形状、有序度的方法,和结晶相通过调整阳极氧化参数和随后的热退火。将介绍阳极 TiO2 纳米管阵列的尺寸和特性之间的关系。简要讨论了阳极TiO2纳米管形成机理研究的最新进展和意义。此外,我们将展示最近在生物医学方向和通过掺杂、表面改性和热退火改进阳极形成的 TiO2 纳米管的性能进行的改性方面最有前途的应用。最后指出了该领域的一些未解决的问题和未来可能的发展方向。
介绍
自二十世纪初以来,二氧化钛 (TiO2) 已被用于防晒剂、油漆、传感器、光催化、太阳能电池、电致变色装置、药物输送等领域的商业生产 [1,2,3,4, 5,6,7]。 TiO2在光照下产生光生电子-空穴对的现象可以帮助将水分解成氧气和氢气,有利于解决未来能源危机,成为最具潜力的燃料。 Fujishima 和他的同事首先报道了在紫外 (UV) 光下在 TiO2 电极上进行光催化水分解 [8,9,10],从那时起,二氧化钛已成为材料科学中研究最多的化合物之一。在所有过渡金属氧化物中,它表现出广泛的功能特性,如化学惰性、耐腐蚀性和稳定性,特别是提高生物相容性 [11] 以及电学和光学性质 [1]。自从饭岛于 1991 年发现碳纳米管 [12] 以来,显示出形状和功能之间的独特组合,其中性质可以直接受到几何形状的影响,纳米技术领域已经做出了巨大的努力,主要是化学、物理和生物医学材料科学。
尽管迄今为止探索最多的纳米材料仍然是碳,但另一类通常基于过渡金属氧化物的纳米管材料在过去 20 年中引起了相当大的兴趣。 Assefpour-Dezfuly [13] 首次尝试形成阳极氧化的二氧化钛纳米管,他使用碱性过氧化物处理,然后在含有铬酸的电解质中进行电化学阳极氧化。由于 Zwilling 等人。报道称,他们于 1999 年在含氟离子的铬酸电解质中通过电化学阳极氧化在 Ti 衬底上制造了第一个自组织纳米管层,该领域得到了极大的扩展 [14]。在过去的十年中,已经发表了 33,800 多篇以“二氧化钛纳米管”为关键词的论文。图 1 给出了 TiO2 纳米管领域每年细分的总出版物,并对 2002-2017 年期间不同合成方法进行了比较,这不仅显示出指数增长趋势,而且显然表明自组织阳极 TiO2 纳米管阵列以巨大的潜力和优势受到广泛关注。最近,李等人。给出了阳极氧化钛纳米管领域的全面和最新观点,几乎涵盖了所有方面,包括生长、改性、性能和应用,并简要介绍了不同的合成方法 [15]。与其他制备方法如水/溶剂热 [16,17,18] 和模板辅助方法 [19, 20] 相比,直接氧化是一种简单的技术,可操作性强,通过调整尺寸获得所需的可控纳米结构,形状和有序度可以通过优化氧化参数(例如施加的电位、时间、温度、pH 值和电解质的组成)来增加 [15]。由于特殊的几何形状,具有高度组织化结构和表面积体积比的自对准氧化物纳米管层表现出独特的特性,例如非常高的机械强度和大的比表面积,甚至提供了诸如高电子的电子特性。迁移率或量子限制效应 [15, 21]。此外,电化学阳极氧化是一种低成本工艺,不仅限于钛,还适用于其他过渡金属 Hf [22]、Zr [23]、Nb [24]、Ta [25]、V [26] 或合金TiAl [27] 和 TiZr [28]。本综述仍将重点放在通过自组织电化学阳极氧化从 Ti 金属基底生长的 TiO2 纳米管上。此外,我们将重点介绍这种自组织二氧化钛纳米管层的合成以及通过调整阳极氧化参数和随后的热退火来影响尺寸、形状、有序度和结晶相的方法,包括四个不同的世代不同于电解质种类和定义的两步阳极氧化等。将介绍阳极 TiO2 纳米管阵列的尺寸和特性之间的关系。简要讨论了阳极TiO2纳米管形成机理研究的最新进展和意义。我们将展示最近在生物医学方向和通过掺杂、表面改性和热退火改进阳极形成的 TiO2 纳米管的性能进行的改性方面最有前途的应用。我们还考虑了该领域未解决的问题和可能的未来发展方向。主要段落文本直接跟在此处。
<图片>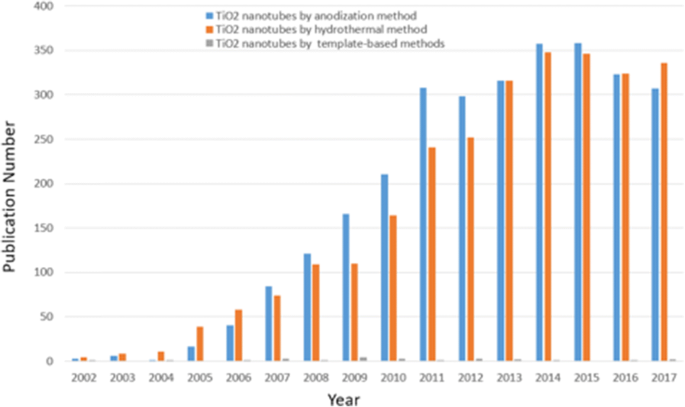
研究趋势。 2002年至2017年与不同合成方法区分的TiO2纳米管相关的每年细分论文数量。(数据来自Science Citation Index Expanded using Titanium Nanotubed,以阳极氧化或水热法或基于模板的方法为关键词)> 图>
电化学阳极氧化合成TiO2纳米管阵列
近年来,虽然已经成功开发了多种形式的纳米结构二氧化钛,包括纳米棒、纳米粒子、纳米线和纳米管 [29,30,31],但纳米管由于其独特的自组装结构而吸引了越来越多的技术应用的兴趣。大的界面面积和方便的尺寸和形状控制,可以作为更好的候选者应用于依赖于表面积的应用。许多优秀的评论 [1, 2, 15, 32,33,34] 可用于处理按不同合成方法分类的 TiO2 纳米材料的特征。电化学阳极氧化被证明是获得二氧化钛纳米管的最有效方法之一,因为它是一种相对简单的技术,可以轻松实现自动化。下面我们将详细说明制备阳极TiO2纳米管的主要技术。
自组织的阳极二氧化钛纳米管阵列
正如广泛研究的那样,二氧化钛纳米管层可以在特定的环境条件下形成。氧化装置由三部分组成: (I) 三电极系统,以制备的钛箔为工作电极,依次在丙酮、乙醇和去离子水中进行超声处理脱脂,铂为对电极,通常为 Ag/AgCl作为参比电极(图 2a),而有时加入 pH 电极以获得 F − 的最终浓度 和 HF [35] 或另一种简单的双电极系统,由 Ti 箔作为阳极和惰性金属电极作为阴极(图 2b)[36]; (II) 一般为含氟离子、氯离子、铬离子、溴离子或高氯酸盐的电解质; (III) 直流电源。影响二氧化钛纳米管应用前景的阳极氧化条件有两个主要特征:(I) 几何形状:尺寸、形状、有序度、结晶相等;(II) 化学、物理和生物医学。换句话说,通过控制电化学阳极氧化参数(施加的电位、阳极氧化的持续时间、电解液系统,包括氟离子的浓度和电解液中的水含量、电解液温度、电解液 pH 值等,这些将更详细地讨论)在“通过电化学阳极氧化合成 TiO2 纳米管阵列”部分)中,可以制造不同的二氧化钛纳米结构,例如扁平致密氧化物 [1]、多孔层 [1, 36]、成束生长的无序 TiO2 纳米管层 [37] ,或最终高度组织的规则 TiO2 纳米管或高级纳米管层:支管 [38]、竹状 [38, 39]、双壁 [40]、纳米花边 [38] 或双层 [39] 结构可以以不同的方式找到属性的方式。图 3 和图 4 显示了此类 TiO2 纳米管形貌典型实例的场发射扫描电子显微镜 (FE-SEM) 图像。
<图片>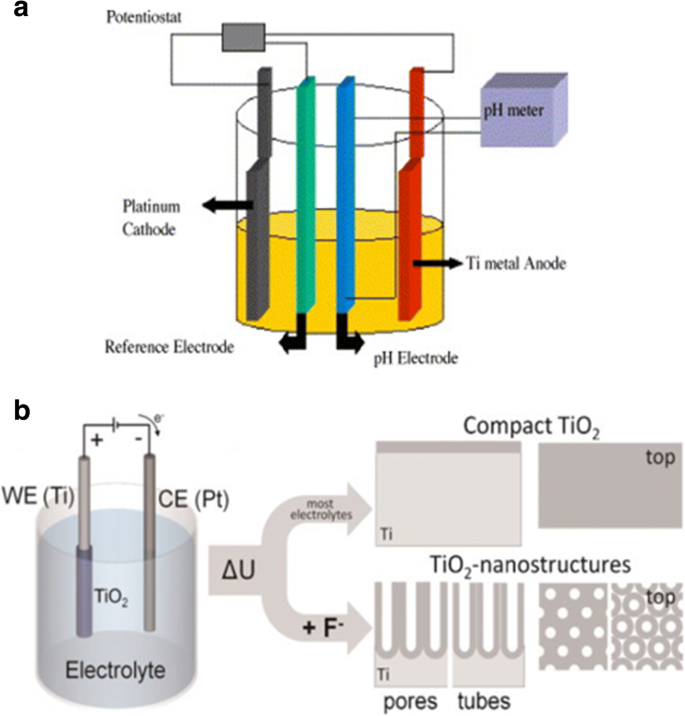
示意图设置。 一 三电极系统示意图,制备的钛箔作为工作电极,铂作为对电极,通常是Ag/AgCl作为参比电极,而pH电极作为pH计。转载自参考。 [35]。 b 由 Ti 箔作为阳极和惰性金属电极作为阴极组成的简单双电极系统的说明图。阳极氧化在不同的条件下会导致不同的阳极氧化层。在大多数中性和酸性电解质中,可以形成致密的二氧化钛。但如果使用稀释的氟化物电解质,纳米管/纳米多孔氧化物层将直接附着在金属表面。转载自参考。 [36]
图> <图片>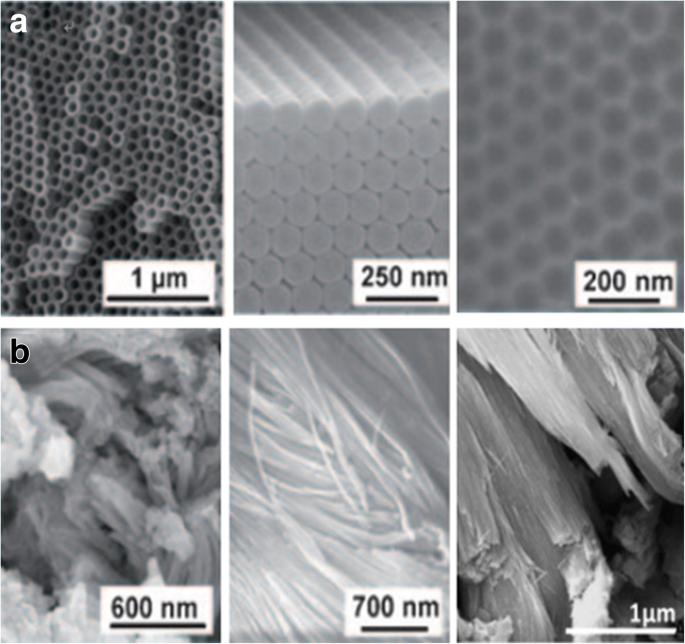
通过 Ti 的不同阳极氧化工艺阳极氧化的 TiO2 纳米管层的 SEM 图像。 一 高度有序的 TiO2 纳米管(顶视图和侧视图)是在有机电解质系统中获得的,具有自序表面凹坑(右),当管层被移除时,这些凹坑实际上是金属表面。转载自参考。 [1]。 b 无序的 TiO2 纳米管在表面区域以补丁形式生长,并通过称为快速击穿阳极氧化 (RBA) 的超快阳极氧化技术在含氯化物的电解质中融合成束。转载自参考。 [1]和[37]
图> <图片>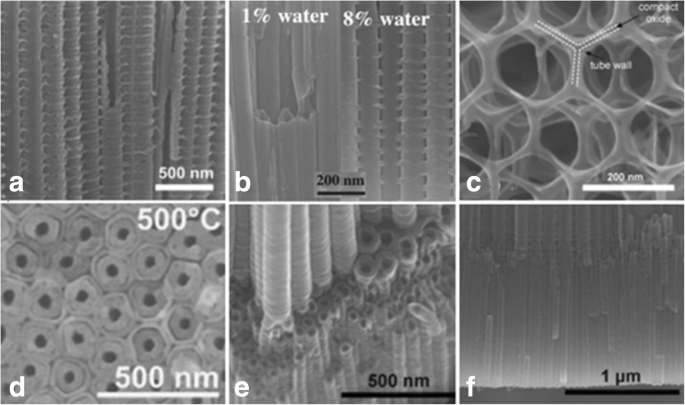
高级 TiO2 纳米管形态的 SEM 图像。 一 在特定的交流电压 (AV) 条件下,在由 0.2 mol/L HF 组成的乙二醇中制造竹型增强 TiO2 纳米管,序列为 120 V 下 1 分钟和 40 V 下 5 分钟。 [38]。 b 0.135 M NH4F/乙二醇电解液中控制加水量(水含量:1%~8%)的阳极氧化可诱导TiO2纳米管从光滑过渡到竹状纳米管
从参考转载。 [39]。 c 2D 纳米线结构是在含氟化物的电解质中进行长时间的电压循环下获得的,序列为 120 V 下 50 秒和 0 V 下 600 秒。 [38]。 d 双壁 TiO2 纳米管是通过 Ti 在含氟化物的乙二醇电解质中在 120 V 下阳极氧化后在 500 °C 下以 1 °C 的加热速率进行阳极氧化而生长的 s -1 .转载自参考。 [40]。 e 可以通过电压步进观察支化纳米管,首先在 120 V(6 小时),然后在 40 V(2 小时)。转载自参考。 [38]。 f 可以看到具有相同或两个不同管径的双层纳米管。转载自参考。 [38]
图>(目前可以得到管径10~500 nm、层厚几百纳米~1000 μm、壁厚2~80 nm的TiO2纳米管阵列[15, 41])
二十年前,增田和福田首次报道了通过将阳极氧化条件调整到最佳状态来获得高度有序的多孔氧化铝[42]。后来,研究人员努力为 TiO2 纳米管层制造类似的组织结构。影响阳极氧化钛纳米管阵列有序度的三个关键因素(根据层中的多边形和管径的标准偏差):钛基板、施加的电压和重复阳极氧化[33, 43] .很明显,在低于介电击穿的最高可能电压下,高纯度材料可以获得较少的排列缺陷 [33],并且如图 5 所示的理想六边形自排序 TiO2 纳米管可以通过二次管生长得到显着改善[43]。索法等人。表明杂质强烈影响第二次阳极氧化后纳米管的不同尺寸和排序[44]。此外,通过电子背散射衍射 (EBSD),Ti 衬底晶粒的晶体取向对 TiO2 纳米管阵列的生长特性具有重要影响。莱昂纳迪等人。发现纳米管只能以能够在晶粒上形成阀金属氧化物的取向观察到,从而允许氟离子穿过氧化膜,其中使用 1 M (NH4)H2PO3 + 0.5 wt% NH4F 作为电解质 [45]。类似地,Macak 及其同事报告说,与使用水性电解质的情况相比,广泛使用的基于乙二醇的电解质中晶粒上的纳米管生长没有受到阻碍,如上一篇文献 [46] 所知。在抛光的 Ti 片上,具有 [0 0 0 1] 取向或接近该取向的晶粒被证明是理想的晶粒,利用具有理想取向的单晶 Ti 将是获得最均匀纳米管阵列的巨大进步 [46] .
<图片>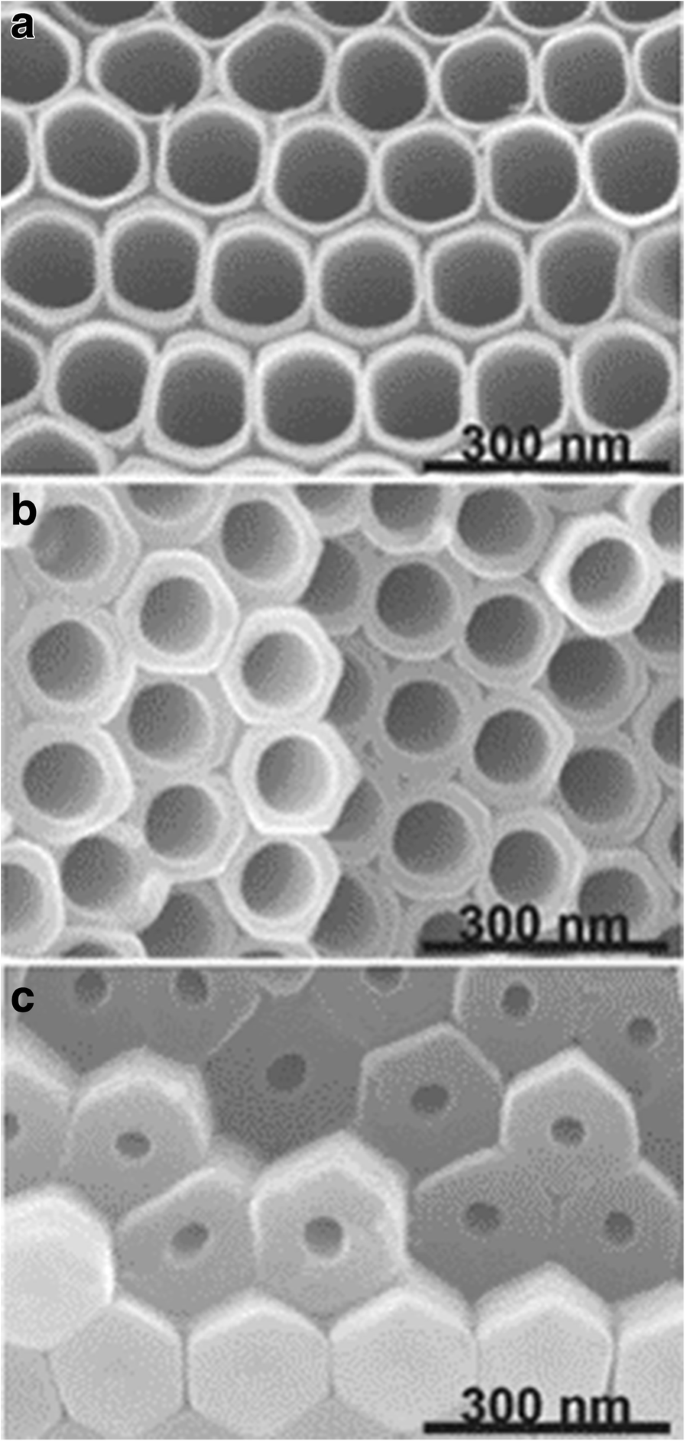
TiO2 纳米管的 SEM 图像。纳米管是在含有 0.27 M NH4F 的乙二醇电解质中通过 Ti 的重复阳极氧化形成的。在层的顶部、中部和底部截取横截面。转载自参考。 [43]
图>尽管如此,仍然存在一些影响有序度的缺陷。最近,它通过均匀的纳米压印 Ti 得到了进一步扩展。近藤等人。通过纳米压印 Ti 表面或顶部有 Al 层、底部有 Ti 层的两层样品,使用具有有序凸起的 Ni 模具,计算出理想有序阳极 TiO2 的产量制造。并且可以以更有序的方式生成 TiO2 层,其中通过随后在 NH4F 乙二醇溶液中进行阳极氧化,预先纹理化图案的浅凹面充当起始点 [47, 48]。紧随其后,Sopha 等人。首先在通过原子层沉积 (ALD) 制备的 Ti 衬底上覆盖 TiN 保护层,然后通过聚焦离子束 (FIB) 进行预纹理化,随后使用乙二醇电解质进行阳极氧化,以产生具有一定厚度的完美六边形排列的纳米管层2 μm,可以限制纳米管只在给定的起始位点上生长,延长阳极氧化时间而没有任何缺陷[49]。
阳极氧化钛纳米管的形成机制
阳极氧化技术和阳极TiO2纳米管形成机理的研究长期以来受到广泛学科的广泛关注。 Diggle 于 1969 年报道的致密阳极氧化膜和多孔阳极氧化膜的机理研究 [50] 现在仍然起着极其重要的指导作用。最近的大量工作表明,从孔到管的过渡是渐进的 [1, 27, 36];但没有给出完整的理论模型和推理。
传统的场辅助溶解 (FAD) 是最可接受的理论 [1, 33, 51]。即在电化学阳极氧化过程中,TiO2纳米管阵列是由二氧化钛自组织形成的,因为三个相对独立的过程:Ti电化学氧化成TiO2、电场诱导TiO2溶解和氟离子诱导化学反应。 TiO2 溶解,达到微妙的平衡。作为图 6 所示的导致纳米管形成的含氟电解质的特征电流时间曲线 [51] 和有助于说明形成过程的典型图像 [33],如图 7 所示,瞬态可以分为分为三个不同的阶段:(I)在第一部分,有一个电流衰减,由新形成的阻挡氧化物引起,当两个主要过程时,O 2− 的向内迁移 离子向金属/氧化物界面和 Ti 4+ 的向外迁移 离子朝向氧化物/电解质界面,达到平衡。 (II) 在第二部分中,由于阳极表面积的增加,电流再次开始上升,并有一个时间滞后。滞后时间越短,由于氟化物诱导形成的 TiO2 溶解,氟化物浓度越高,孔隙开始随机生成,随后证明是 TiO2 纳米管的初始形成。 (III) 然后,当金属氧化物界面处的孔隙增长速率和外界面处形成的 TiO2 的诱导溶解速率达到平衡状态时,电流达到稳定状态。因此,最终的管子变得越来越 v 形,也就是说,管子的顶部具有比管子封闭填充的底部明显更薄的壁。图 5 中管壁厚度的梯度可归因于不同的暴露时间和沿管的电解质浓度 [43]。
<图片>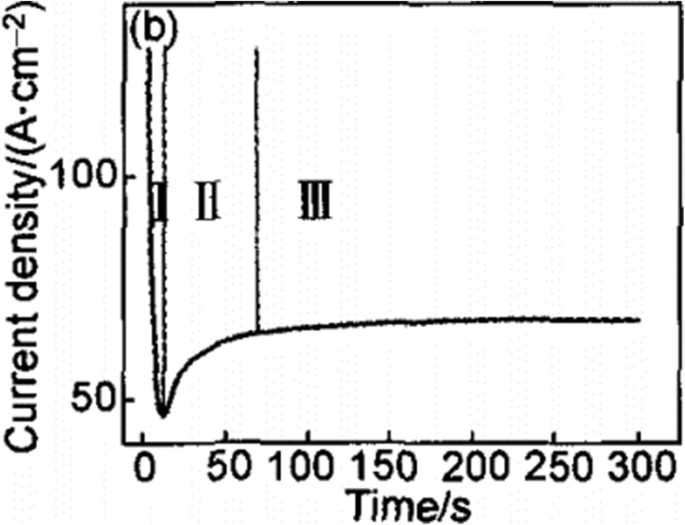
在含氟电解质中恒压下的典型电流时间曲线。瞬态可以分为三个不同的区域(I –三 )。 (我 ) 在第一部分,有一个急剧的电流衰减。 (二 ) 在第二部分,电流开始再次上升,但有一个时间滞后。 (III ) 在第三部分中,电流达到了从参考再现的稳定状态。 [51]
图> <图片>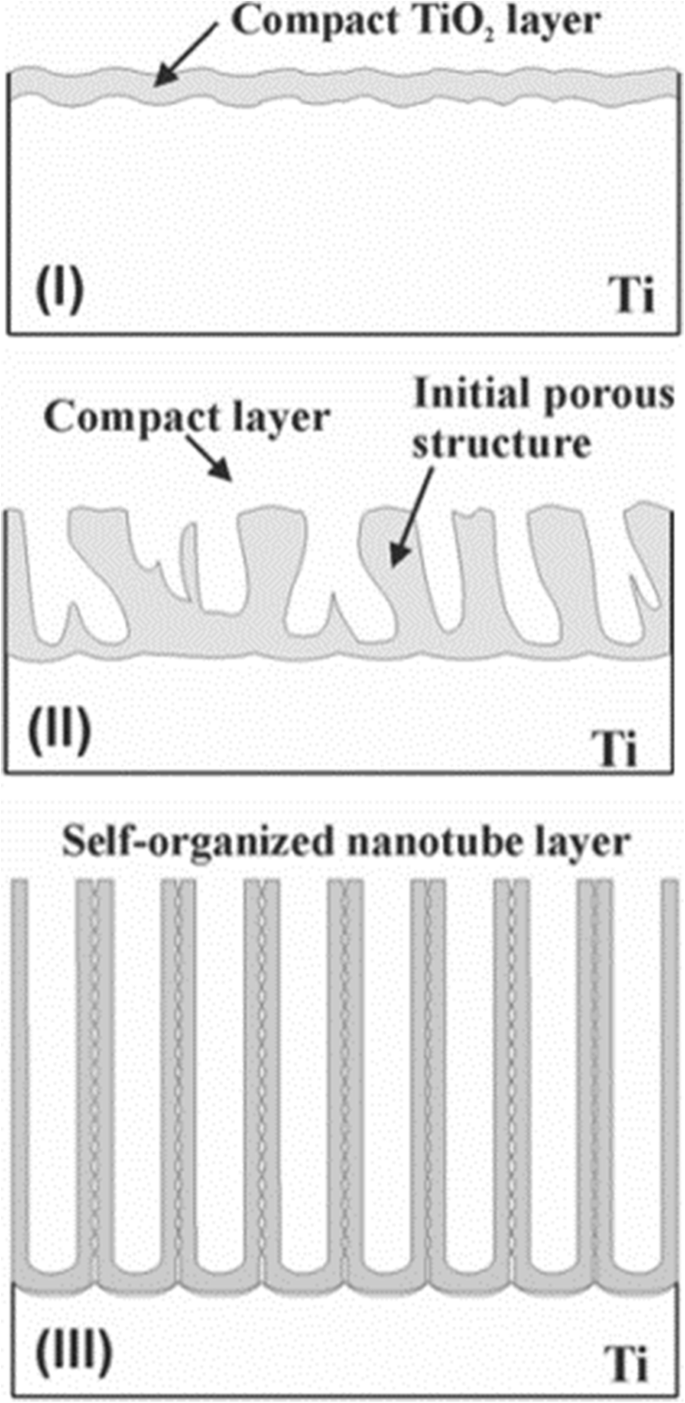
TiO2纳米管阵列的形成过程。 TiO2纳米管阵列的形成可分为三个不同的形态阶段(I –三 )。 (我 ) 形成阻挡氧化物。 (二 ) 表面被局部激活,孔隙开始随机生长。 (III )自组织纳米管层形成自参考文献。 [33]
图>然而,该理论无法解释分离成管的现象,而不是清楚地与纳米多孔结构相反,Fahim 等人。观察到,在适当的电压下,可以在不含氟离子的硫酸溶液中获得二氧化钛纳米管,在这种情况下,I-t 曲线类似于我们刚才讨论的曲线 [52]。正如 Houser 和 Hebert 所指出的,尚未开发出生长机制来解释二氧化钛多孔膜过程与 I-t 曲线之间的定量关系[53]。由于解释不够有说服力,最近出现了关于该机制的新观点,如粘性流模型和两流增长模型。针对这些机制,综述[51]指出了传统场辅助溶解理论的诸多局限性,并对两种流粘性流模型和增长模型研究的最新进展和意义进行了一些解释。
阳极氧化条件对几何形状和性能的影响
电解质的组成和浓度对纳米管阵列的形成有显着影响。根据我们使用的电解液的不同,发展基本分为三个阶段:表1总结了迄今为止不同研究组研究的三代TiO2纳米管阵列的阳极氧化条件和尺寸。
图>第一代:氢氟酸(HF)基水系电解质
里程碑是龚等人。首次通过在 HF 基水性电解质中对 Ti 进行阳极氧化来呈现均匀的二氧化钛纳米管阵列 [54]。在 HF 水溶液电解质中,pH 值相对较低意味着氢离子浓度较高,氟离子引起的 TiO2 化学溶解在阳极氧化过程中起主导作用 [55]。二氧化钛纳米管的形成过程在短时间内达到了动态平衡,因此纳米管的最大长度限制在0.5 μm左右[54,55,56]。
第二代:缓冲电解质
在随后的工作中,为了减少加长管的化学溶解,蔡等人。证明通过将较弱的酸(如 KF 或 NaF)加入缓冲溶液中,并用硫酸或氢氧化钠将 pH 值调节至弱酸性(pH =4.5),可以获得长度约为 4.4 μm 的纳米管 [57]。 PH 值影响钛离子的水解,结果证明会干扰电化学蚀刻和化学溶解。蔡等人。还指出,较低的 pH 值会产生较短但干净的纳米管,较高的 pH 值会导致较长的纳米管但会产生不需要的碎片 [57]。随着 pH 值升高,水解速率会增加,进而减缓化学溶解,导致纳米管更长,而碱性溶液不适合纳米管生长 [57, 58]。 Macak 等人证明,在中性 NaF 电解质中,在适当的电压下,可以获得比酸性溶液中更长的纳米管。 [58]。在含氟电解质中给定特定电压,通过调节 pH 梯度,可以获得所需的纵横比和层厚度 [59]。
第三代:极性有机电解质
甘油[59]、二甲亚砜[60]、甲酰胺或二甘醇[61, 62]、乙二醇[41, 63]等含有NH4F、NaF、KF等氟化物的电解质逐渐出现。 Macak 及其同事率先使用粘性甘油电解质制造了厚度约为 7 μm、平均管径为 40 nm 的二氧化钛纳米管阵列 [59]。结果表明,由于适当控制电解质 pH 值可减少二氧化钛的化学溶解,因此可以在这种极性有机电解质中生长更高纵横比的 TiO2 纳米管 [64]。保罗斯等人。形成的纳米管长约 134 μm,使用含有 0.25 wt% NH4F 的乙二醇在 60 V 阳极氧化电位下制备 17 小时 [60]。不久之后,Albu [65] 报道了超过 250 微米厚的 TiO2 纳米管阵列。此外,水分在该过程中起着双重作用:它对于二氧化钛的形成是必不可少的,但它也加速了化学溶解[63]。因此,增加TiO2纳米管阵列的厚度和有序度需要如何将含水量的影响减小到最小的显着性。一般来说,将水含量限制在 5% 以下是成功实现超长纳米管的关键 [60],并且需要最少的水含量 (0.18 wt%) 来形成组织良好的二氧化钛纳米管 [66]。据报道,随着水的加入,记录的电流密度降低,在无水乙二醇溶液中最高[66]。保罗斯等人。首次报道了在含有 0.6 wt% NH4F 和 3.5% 水的乙二醇中在 60 V 下形成长度约为 1000 μm 的自组织六边形二氧化钛纳米管阵列 216 小时 [41]。另一个值得注意的现象是光滑的管壁在低含水量下生长,而侧壁上的波纹在高含水量下形成,如图 4b [59, 67] 所示。作为迄今为止最常用的电解质类型,含有水和氟离子的乙二醇总是导致双壁纳米管结构(图 4d)[40, 68,69,70],而内层可以通过适当的退火处理,然后是简单的化学蚀刻工艺。去除内壳后,加宽的管允许使用基于 TiCl4 水解的重复方法用纳米粒子逐层装饰 [71]。而单壁管在染料敏化太阳能电池 (DSSC) [71, 72] 中显示出显着增强的电导率和电子传输时间,其中整个管的厚度基本相同并且不再出现内壳,Mirabolghasemi 等人。对双壁管和单壁管进行了比较,并提出了在含有 1.5 M H2O 和 0.1 M NH4F 的电解质中添加二甲基亚砜 (DMSO) 的所需单壁管 [72]。
最近,据报道,非氟化物基电解质可以生长 TiO2 纳米管阵列,这可以被认为是第四代合成材料,包括盐酸、过氧化氢、高氯酸溶液及其混合物 [73, 74]。 Allama 和 Grimes 描述了在 10 到 13 V 的氧化电压下在 3 M 盐酸 (HCl) 水溶液电解质中获得长度为 300 nm、内径为 15 nm、外径为 25 nm 的发达的纳米管阵列。 但是添加低浓度的 H3PO4 导致从纳米管变为棒状。他们进一步提出,在低于或高于 3 M 的浓度下,他们无法在含 HCl 的电解质中实现自组织纳米管阵列 [73]。 Allama 发现在含盐酸的水溶液中加入过氧化氢可能是一种延长二氧化钛纳米管的可能方法,二氧化钛纳米管在较厚的氧化层后具有强氧化性,表明氟离子可以成功地在生长过程中被氯离子取代纳米管阵列[74]。此外,近年来,不添加游离氟化物的离子液体作为另一种类型的二氧化钛纳米管溶剂体系被处理[75, 76]。
除了标准参数外,所得纳米管的几何形状还取决于电解质的重复使用(“使用过的溶液效应”)。与用新鲜溶液获得的管相比,使用一次使用过的溶液显示出纳米管长度的增加和更好的质量,其中在 60 V 及更高电压下使用一次的溶液获得的纳米管生长速率始终较高 [77] 和可以注意到略有不同但可区分的电流瞬态行为 [66]。此外,由于 F - 的耗尽,在两次使用的溶液中没有获得纳米管结构,而是获得了氧化膜 种 [78]。然而,Sopha 等人。研究了不同年龄的乙二醇基电解质对 TiO2 纳米管形态的影响,结果表明,在较旧的电解质中,阵列表现出较低的纵横比 [79]。
应用潜力
阳极氧化电压是控制管直径的关键因素 [80, 81]。纳米管阵列的尺寸可以简单地通过在电极上施加称为电位窗口的合适电压范围来预测 [67]。在低电压下,发生较少的电场溶解,形成直径较小的 TiO2 纳米管。如果电压太低,TiO2 层会变得致密,但无法观察到纳米管状结构。相反,当电压过高时,会看到海绵状的多孔结构。在可控电压下,纳米管的直径与电压成正比 [81]。此外,研究表明,形成纳米管的电压范围也与电解质体系有关。在水性电解质中,电位窗口应控制在 10 到 25 V 之间,而在有机电解质中,电位窗口在几伏到几百伏之间要宽得多。 Wang 和 Lin 发现,在水性电解质中,阳极氧化电位对 TiO2 纳米管阵列的生长有显着影响,在这方面,在非水性电解质中表现出轻微影响 [82]。非水电解质的电压依赖性显着降低,这在很大程度上归因于有机电解质的低电导率[83, 84]。
The Duration on Anodization
The duration of anodization affects the nanotubes mainly in two aspects:(I) the formation of the tubes or not and (II) the length of the tubes. That is, in the early stage of the anodization, a compact TiO2 film is formed. If the duration is too short to reach an equilibrium in reaction, the regular nanotube array cannot be achieved instead of a disordered porous layer [67]. With increasing the anodization time, porous structure gradually grows deeper and converts into the TiO2 nanotubular array [1, 33, 51]. If other electrochemical parameters are kept unchanged, increase in the nanotube length is observed over time while no significant effect on diameter and tube wall thickness until a steady-state situation occurs [67, 85, 86]. However, due to the decrease of the F − concentration in the electrolyte, where the ion transport rate decreased, the growth rate of nanotubes is reduced. After reaching a stable condition between tube growth at the bottom and chemical/electrochemical dissolution at the top, we will find no further increase in length of the nanotubes [87]. As time continues to go, pipe orifice becomes an irregular polygon resulting in TiO2 spikes and coverings which can be seen on the surface of the TiO2 nanotube arrays [36]. It is worth mentioning that enlightened by the success of aluminum-repeated anodization for self-organized porous alumina [88], the two-step anodization of titanium for such a highly ordered hexagonally packed nanostructure of titania has appeared [43, 77, 89,90,91]. After the first-step anodization, the first nanotube layer from the Ti foil should be removed ultrasonically or by using an adhesion tape which leads to a surface where the remaining Ti is covered by comparably ordered dimples. Researches have shown that the former treatment helps to avoid potential mechanical damage to the Ti surface and also improve the structural uniformity of the TiO2 nanotubes to a great extent [77, 90, 91]. In the second anodization step, the pretreated Ti foil would be used as anode again with or without changes in parameters of oxidation conditions. It is subsequently found that the highly ordered and vertically oriented titania nanotubes, have greater potential in such fields as photocatalysis [77], photoelectriochemical activity [92, 93], and biological interaction with cells [94] than the disordered nanotubular titania.
Electrolyte Temperature
Temperature restricts the growth and quality of titania nanotube arrays, directly affecting the rate of oxide growth, length, and wall thickness of the structure [64, 95]. Wang and Lin first reported the effect of electrolyte temperature in both aqueous and non-aqueous electrolyte on anodic oxidation of titanium [82]. In aqueous electrolyte, with the temperature increasing, a slight diminish in the internal diameters was observed while the external diameters remained the same [68]. The reason may be the dissolution induced by electrical field and fluoride ions are similar while the oxide formation rate is higher than that at lower temperature. In non-aqueous electrolyte containing fluoride ions, the outer nanotube diameter was found to be largely increased by the increasing electrolyte temperature [82]. This may be because at lower temperature, the ion mobility of fluorine in some viscous electrolyte is further inhibited, leading to much slower dissolution of newly formed titania, which subsequently lead to a smaller nanotube diameter. As chemical dissolution rate increases, surface of TiO2 nanotubes arrays can easily produce excessive corrosion, resulting in lodging nanotubes and agglomeration. Therefore, the appropriate bath temperature for stable TiO2 nanotube arrays is at room temperature [82, 95, 96].
Modification of Nanotubes Properties
Increasing applications of TiO2 nanotubes as a novel semiconductor are closely related to its photoelectriochemical (PEC) performance; however, they are sometimes prevented by two fundamental drawbacks:(I) the wide band gap (3.0 eV for the rutile phase and 3.2 eV for the anatase phase) can only absorb ultraviolet light, which accounts for less than 10% of the sunlight [97], resulting in low average utilization ratio of solar energy and (II) the low electrical conductivity cannot efficiently transfer photogenerated carries. At the same time, the photoelectrons and vacancies can be easily recombined, thus making low electron mobility rate or quantum confinement effects [98]. Hence, post-treatment of TiO2 nanotubes is the key to improve the performance of its materials and related devices successfully. Considerable researches have been reported on modified methods to reduce the recombination of photogenerated electron-hole pair rate, speed up the electron transfer rate, and enhance the photoelectriochemical activity of TiO2 nanotubes. The research of the methods for the improvement of the photoelectriochemical properties of TiO2 nanotubes will be reviewed, including thermal annealing, doping, and surface modification. As for promising modification in biomedical fields, we will present in the application section.
Thermal Annealing
The crystallinity of the nanotube arrays and their conductivity, lifetime of charge carrier, and photoresponse depend mainly on the thermal annealing temperature and atmosphere [99, 100]. The as-prepared TiO2 nanotubes above are amorphous in nature but can be annealed to anatase or rutile phase, or mixtures of both phases relying on the specific temperature [1, 3, 40, 92, 100]. It is demonstrated that amorphous nanotube layers grown in a glycerol-based electrolyte containing fluoride ions have low photocurrents and an incident photon-to-electron conversion efficiency (IPCE) below 5% due to lots of structural defects while anatase phase nanotubes exhibit an IPCE value up to 60% thus attracting more interest to applications such as dye-sensitized or perovskite solar cells [93]. As well in mixed water-glycerol electrolyte with F − , Das et al. stated their points that if the self-organized TiO2 nanotube arrays with thickness about 1 μm were annealed around 300–500 °C, the anatase phase of TiO2 as the most preferred crystalline structure could be observed. The single anatase structure of nanotubes with the best photoelectriochemical properties and the lowest resistivity could be fabricated when annealed at 400 °C. At temperature higher than 600 °C, a track of typical rutile appeared and with a further increase in annealing temperature the percentage and quality of the rutile phase increased [92]. It should be noted that in Jaroenworaluc’s work, rutile phase was detected in anodic nanotube layers grown in aqueous NaF/Na2SO4 with thickness of approximately 1.5 μm at 500 °C heat treatment and became the dominant phase at 600 °C. Whereas at 550 °C, partial nanotubes began to break down [101]. It begins to cause the collapse of the entire nanostructure formed in aqueous NaF/Na2SO4 with the continuous increase of temperature (800–900 °C) or the extended annealing time [3]. While for extended temperature, the crystalline structure of the nanotubes completely converts to rutile phase at above 900 °C [3]. Some researchers demonstrated a loss of the typical single-walled nanotube layers morphology when the annealed temperature rose above 580 °C [102]. Besides the whole annealing process especially the heating rate controls, the morphological structures of the entire nanotube arrays [40]. The double-walled nanotube layers prepared from ethylene glycol (containing less than 0.2 wt% H2O), with the addition of HF and H2O2, have such a high stability that can keep their structure intact until temperature is higher than 900 °C with a heating rate of 1 °C s −1 . However, the double-walled nanotubes begin to collapse as soon as the temperature reaches 500 °C when the heating rate is 25 °C s −1 . Most extraordinarily, with the high speed of 50 °C s −1 the entire separated nanotubes fuse into a highly ordered porous membrane [40]. Xiao et al. obtained crystallized titania nanotubes arrays with calcination in different gases like dry nitrogen, air, and argon indicating nanotubes in dry nitrogen appeared to have enhanced electrochemical and photoelectrical properties who also found out that with the increasing temperature internal diameter decreased while wall thickness increased at the expense of nanotubes length [103].
As shown in Fig. 8, the conductivity along the TiO2 nanotubes with three different thickness is strongly affected by annealing temperature. Smallest resistance is observed at about 350–450 °C when the amorphous nanotube arrays are totally converted into anatase layers [99]. And it is evident to see that specific resistivity increases with thicker nanotube arrays which can be shown more clearly in the inset in Fig. 8. Furthermore, calcination temperature is responsible for the decrease in the length of the anatase TiO2 nanotubes. As shown in Fig. 9a, increment of temperature between 300 and 500 °C causes the as-prepared nanotube arrays slightly changing in thickness from 13.6 to 12.6 μm. When annealing temperature continuously increases to 600 °C, the average length of the nanotubes decrease dramatically to 6.6 μm. Figure 9b shows conversion from anatase TiO2 to rutile phase TiO2 occuring at 500 °C when the rutile barrier layer is formed on the bottom of the TiO2 nanotube arrays along the anatase nanotubes by consuming the bottom layer if the annealed temperature is further increased. This leads to a length decrease and corresponding photocatalytic activity decline [104].
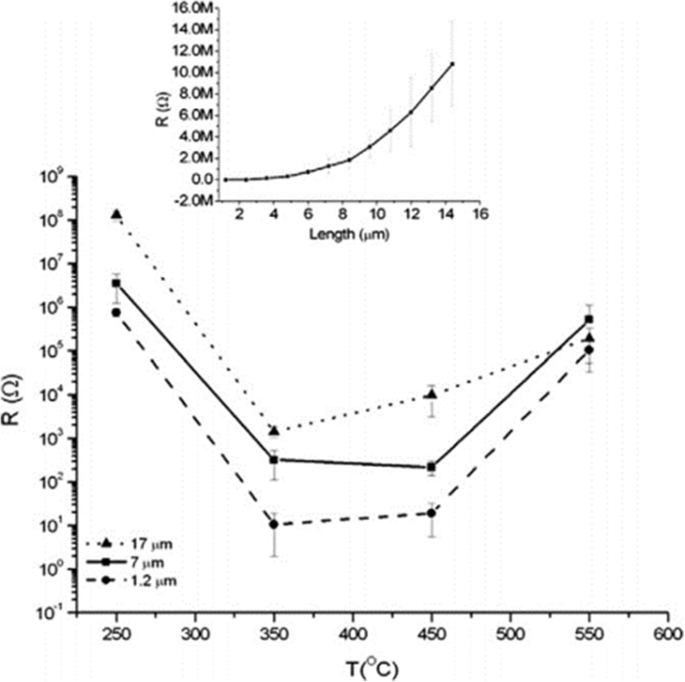
Electrical resistance as a function of the annealing temperature for the different nanotube layer thicknesses. The curve shows electrical resistance measurement for different titania nanotube arrays grown in ethylene glycol based electrolyte containing HF and water at different temperature and the influence of thickness on resistance. The inset shows more details about the relationship between the thickness of the nanotube arrays annealed at 250 °C and their specific resistivity. Reproduced from ref. [99]
图>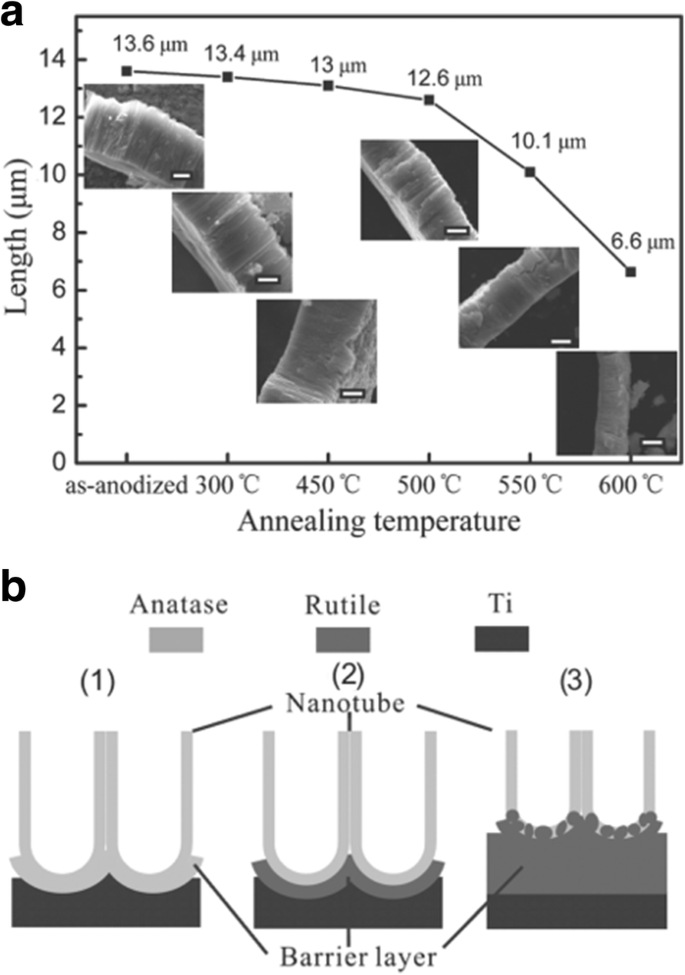
Evolution of titania nanotube arrays at different calcination temperatures. The electrolyte was ethylene glycol containing 0.3 wt% ammonium fluoride and 5 vol% distilled water. 一 The decrease in the thickness of titania nanotube arrays at different annealing temperature from 300 to 600 °C. The insets are corresponding SEM images and the scale bar is 5 μm. b The schematic of crystallization process of anodic titania nanotubes annealed at (1) 450 °C, (2) 500 °C, and (3) 600 °C in air. Reproduced from ref. [104]
图>Doping
Doping ions or atoms into titania lattice, a substitution within the lattice either at Ti 4+ 或 O 2− sites, on the one hand, changes the lattice constants and bond energy. On the other hand, it is beneficial to the separation between photogenerated electron and hole pair, which in turn adjusts the band gap and improves the photoelectrochemical performance of nanotubes [15]. The impurity doping has been commonly applied to extend the light absorption onset of TiO2 nanotubes by either introducing subbandgap states or adjusting its bandgap width [105]. Lately, co-doping approach has been proposed as a more efficient way to reduce the band gap and adjust energy band level in favor of photoelectriochemical reactions [106, 107]. There are various kinds of doped-elements and preparation methods, and Table 2 summarizes some methods and the doping effects of doped titania nanotubes.
图>The most typical doped TiO2 nanotubes are as follows:
- i.
Metal-doped TiO2 nanotubes such as Nb [107], Fe [108], Cu [109], Cr [110], Zr [111], Zn [112], and V [113]
- ii.
Non-metal-doped TiO2 nanotubes such as N [105], F [114], B [115], C [116], S [117], and I [118]
- iii.
Co-doped TiO2 nanotubes such as N–Ta [105], N–Nb [107], and C–N–Ni [119]
Choiet systematically studied the photoreactivities of 21 metal ion-doped quantum-sized TiO2 doping with Fe, Mo, Ru, Os, Re, V, and Rh significantly increases quantum efficiency, while Co and Al doping decreases the photoreactivity [120]. Momeni et al. recently obtained Fe-TiO2 nanotube (Fe-TNT) composites using different amounts of irons to decorate anodically formed TiO2 nanotubes with potassium ferricyanide as the iron source, indicating that Fe doping efficiently accelerates the photocatalytic performance for water splitting [108]. Not limited to transition metals, other elements including N [105], F [114], B [115], C [116], S [117], and I [118] are successfully explored. Nitrogen-doped TiO2 nanotubes turns out to be a promising path to narrow the band gap energy with enhanced photocurrent response in the visible light and the tube length influences the magnitude of conversion efficiency [121, 122]. Kim and co-workers proved that TaOxNy layer-decorated N-TNT (N-doped TiO2 nanotubes) as dual modified TNTs have significantly improved both visible (3.6 times) and UV (1.8 times) activities for water splitting [105]. At present, more researches are aimed at co-doping which exhibits remarkable synergistic effect causing a significant improvement on photoelectriochemical properties. Chai et al. grew Gd–La co-doped TiO2 nanotubes by an ultrasonic hydrothermal method, enhancing visible light photocatalysts [123]. Cottineau et al. modified titania nanotubes with nitrogen and niobium to achieve co-doped nanotubes with noticeably enhanced photoelectriochemical conversion efficiency in the visible light range [107]. Nevertheless, the mechanism for increasing photoconductivity and synergistic effect of various elements on co-doping remains a further study.
Surface Modification
Surface modification means decoration on surface of TiO2 nanotube arrays with nanoparticles (metal, semiconductors, and organic dyes). Nanowire arrays can also be fabricated by electrodeposition into titanium oxide nanotubes [124]. TiO2 nanotube is a semiconductor with a wide band gap, which can only absorb ultraviolet light [97, 125]. Any other nanomaterials which possess a narrow band gap or can absorb the visible light can be used as a sensitizer for titania nanotubes. Silver nanoparticles can be decorated on the tube wall by soaking the titania nanotube arrays in AgNO3 solutions and photocatalytically reducing Ag + on a TiO2 surface by UV illumination [126]. Ag/TiO2 nanotubes show a significantly higher photocatalytic activity and good biological performance compared with neat TiO2 nanotubes [126, 127]. Some compositions such as graphene oxide GO [128], CdS [129], CdSe [130], and ZnFe2O4 [131]. can be modified on TiO2 nanotube arrays. Lately, GO have attracted much scientific interest in nanoscale devices and sensors which is easy to combine with nanostructure materials to compose some compounds. Titania nanotubes fabricated by anodization in water-ethylene glycol electrolyte consisting of 0.5 wt% ammonium fluoride (NH4F) can be incorporated with GO by cyclic voltammetric method, which achieve higher photocatalytic activity and more effective conversion efficiency (GO-modified vs pure nanotubes =26.55%:7.3%) of solar cell than unmodified TiO2 nanotubes [128]. Semiconductor composite is a method improving the performance of titania nanotubes via, in some specific way, combining two kinds of semiconductors with different band gap [132].杨等人。 decorated CdSe nanoparticles on the surface of TiO2 nanotubes by applying an external electric field to accelerate CdSe nanoparticles in nanochannels resulting in a material with more stable and higher photoresponse to visible light. Furthermore, the degeneration rate of anthracene-9-carbonxylic acid when exposed to the green light irradiation indicating that CdSe dominates the photocatalytic process under visible light [130].
Besides, other oxide nanoparticle deposition such as WO3 [133] or TiO2 [134] onto TiO2 nanotubes by the hydrolysis of a chloride precursor also turns out to augment the surface area and improve the solar cell efficiency. Another very effective approach is to consider organic dyes as sensitizers for TiO2 nanotubes to improve its optical properties [135]. Lately, atomic layer deposition (ALD) becomes an established procedure to modify TiO2 nanotube layers. ALD appears to be a very uniform and precisely controllable deposition process to functionalize nanotubes in conformably coating the surface of the nanotube layers with one atomic layer after another of a secondary material, such as Pd [136], ZnO [137], Al2O3 [138], CdS [139], or TiO2 [140].
Biomedical Applications
Historically, the mentioned milestones were reported on the fabrication of titania nanotube arrays contributing to widen the promising applications over the past 20 years in the areas ranging from anticorrosion, self-cleaning coatings, and paints to sensors [141,142,143], dye-sensitized and solid-state bulk heterojunction solar cells [144,145,146], photocatalysis [147, 148], eletrocatalysis, and water photoelectrolysis [149, 150]. They also outperform in biomedical directions as biocompatible materials, toward biomedical coatings with enhanced osseointegration, drug delivery systems, and advanced tissue engineering [15, 135, 141, 142, 151]. In the following section, we will give an overview of current efforts toward TiO2 nanotubes biomedical applications. Titania nanotubes possess good biocompatibility as they show some antibacterial property, low cytotoxicity, good stability, and cytocompatibility including promoting adhesion, proliferation, and differentiation of osteoblast and mesenchymal stem cells (MSCs) with a high surface area-to-volume ratio and controllable dimensions [152,153,154,155].
However, Ti products have inadequate antibacterial ability and efforts have been made to improve their antibacterial properties such as modifications on titania nanotubes for biomedical applications like bioimplant [126, 156].
Biological Coatings And Interactions with Cells
A number of in vitro and in vivo studies have demonstrated that MSCs, osteoblasts and osteoclasts show size-selective response which means the effect of size holds an important position in cell interaction where the optimized size for cell adhesion, proliferation, growth, and differentiation is ranging from 15 to 100 nm [153, 157, 158]. Particularly, it was demonstrated that the TiO2 nanotubes with a diameter of 70 nm was the optimal nanoscale geometry for the osteogenic differentiation of human adipose-derived stem cells (hASCs) [159].史密斯等人。 reported increased dermal fibroblasts and decreased epidermal keratinocyte adhesion, proliferation, and differentiation on TiO2 nanotube arrays (diameter 70–90 nm, length 1–1.5 μm) [160]. As shown in Fig. 10, Peng et al. found that nanotubular surface preferentially promoted proliferation and function in endothelial cells (EC) while decreased in vascular smooth muscle cell (VSMC) by measuring EdU, a thymidine analog which is incorporated by proliferating cells [161]. Furthermore, it is pointed out that surface wettability of the TiO2 nanotube layers is recognized as a critical factor for cell behavior which can be adjusted by changing the diameter of the nanotubes. That is to say, water contact angles can be altered without changing the surface chemistry [158]. To get further understanding of the effect of TiO2 nanotube layers to bone-forming cells as well as stem cells response, Park et al. seeded green fluorescent protein-labeled rat MSCs on TiO2 nanotube layers with six different diameters (15, 20, 30, 50, 70, and 100 nm), resulting in cell activity that is sensitive to nanoscale surface topography with a maximum in cell activity obtained for tube diameters of approximately 15–30 nm. Such lateral spacing exactly corresponds to the predicted lateral spacing of integrin receptors in focal contacts on the extracellular matrix, forcing clustering of integrins into the closest packing, resulting in optimal integrin activation. While tube diameters larger than 50 nm, severely impaired cell spreading, adhesion, and spacing of 100 nm may lead to the cell apoptosis [94]. Besides adjusting the size of the nanotubes, surface modification loaded with bioactive factors should be highlighted, in which case biomedical properties can be further optimized. In the case of bone implants, hydroxyapatite (HA) formation is important for osseointegration. Recent works have shown hydroxyapatite nanocrystalline coating onto the nanotubular TiO2 results in further enhanced osseointegration with strong adhesion and bond strength, and a drastic enhancement of deposition rate is observed [162, 163]. Nanotubular TiO2 surface can greatly enhance the natural apatite growth rate in simulated body fluid (SBF) compared with flat surfaces [10, 164]. The alkaline-treated TiO2 nanotubes with NaOH solutions are more bioactive in SBF, where sodium titanate can significantly accelerate nucleation and the growth of HA formation presenting a well-adhered bioactive surface layer on Ti due to its larger surface area and promoted mechanical interlocking between HA and TiO2 nanotubes [165, 166]. Electrodeposited with hydroxyapatite, higher adhesion of TiO2 nanotubes has been described in the literature by means of adhesive tape test and the live/dead cell staining study which is essential for early bone formation [166]. The results also showed that at the length of 560 nm the highest adhesion of HA surface on the nanotubes is observed. Also the nanotube surface can indeed strengthen Collagen type I expression in vivo experiment which is considered to be a basic initial bone matrix protein in bone formation [167]. Moreover, annealing of the amorphous nanotubes to anatase or a mixture of anatase and rutile was found to be an important factor in the apatite formation process [164].
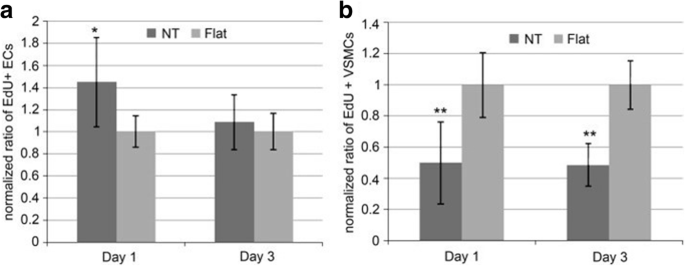
Ratio of EdU positive a ECs and b VSMCs on flat or nanotube substrate. It is normalized by the average proportion of positive cells on flat surfaces on day 1 and 3. Data is presented as average ± standard deviation. *p < 0.05, **p < 0.01 versus same day flat control, n = 6 reproduced from ref. [161]
图>Drug Delivery and Antibacterial Ability
Furthermore, the tubular nature of TiO2 in biomedical devices may be exploited as gene and drug delivery carriers with living matter due to its high surface area, controllable pore, and self-ordered structure [1, 15]. When the orthopedic bioimplant is placed into the bone defect, persistent and chronic infection is one of the most common and serious complications associated with biomedical implantation [16, 168]. Certain dimension and crystallinity may be useful to prevent bacteria adhesion and promote bone formation. The thermal annealing has decreased the number of bacteria adhering to the Ti surface. It could be in part because heat treatment removes the fluorine content which has a tendency to attract bacteria. The research also indicates that nanotubes with 60 or 80 nm in diameter decrease the number of live bacteria as compared to lower diameter (20 or 40 nm) nanotubes [169, 170].
Bauer et al. loaded epidermal growth factor (EGF) and bone morphogenetic protein-2(BMP-2) onto the TiO2 nanotubes surface by covalent attachment. They observed positive influence on the behavior of MSCs on 100-nm nanotube arrays where cell count was at much higher levels compared to the untreated one [171]. Lately, titania nanotubes loaded with antibiotics contribute to suppressing bacterial infections. As gentamicin sulphate (GS) is mostly widely used with highly water solubility, Feng et al. loaded titania nanotubes with GS through physical adsorption and cyclic loading which can treat many types of bacterial infections [172].张等人。 fabricated titania nanotubes loaded with vancomycin to investigate the increasing biocompatibility and obvious antibacterial effect on Staphylococcus aureus [173]. However, systemic antibiotics in clinical will bring many side effects. The release of antibiotics from the nanotubes is too fast to maintain the long-term antibacterial ability, and the use of antibiotics may develop resistant strains [126, 168, 174]. Ensuring a constant release rate becomes a crucial but difficult part in the field of drug delivery. In strategies like surface modification, controlling the dimension of nanotube arrays, biodegradable polymer coating have been employed to solve the issue [21]. Drug release of several drugs such as antibiotics or growth factors from titania nanotube arrays can be adjusted by varying their diameters and lengths [152, 175, 176].冯等人。 covered a thin film comprising a mixture of GS and chitosan on GS-loaded titania nanotubes and showed a controlled release of the drug providing sustained release effects to a certain extent [172]. Titania nanotube arrays as drug nanoreservoirs on Ti surface for loading of BMP-2 were fabricated by Hu et al. and then further covered with gelatin/chitosan multilayers to control the release of the functional molecule meanwhile maintain the bioactivity for over 120 h via a spin-assisted layer-by-layer assembly technique which is mainly based on electrostatic interactions between polyanions and polycations as well as promote osteoblastic differentiation of MSCs [177].赖等人。 successfully fabricated Chi/Gel multilayer on melatonin-loaded TiO2 nanotube arrays to control the sustained release of melatonin and promote the osteogenic differentiation of mesenchymal stem cells [178]. Karan et al. synthesized titania nanotubes loaded with the water-insoluble anti-inflammatory drug indomethacin and modified lactic-co-glycolic acid on surface as a polymer film in order to extend the drug release time of titania nanotubes and produce favorable bone cell adhesion properties, with reduced burst release (from 77 to> 20%) and extended overall release from 4 days to more than 30 days [152]. As previous study reported that surface treatment of implants with N -acetyl cysteine (NAC) may reduce implant-induced inflammation and promote faster bone regeneration [179], Lee et al. examined the feasibility of N -acetyl cysteine-loaded titania nanotubes as a potential drug delivery system onto an implant surface, and the data indicates the enhanced osseointegration and the value of the small animal model in assessing diverse biological responses to dental implants. Besides, TiO2 nanotube arrays are suitable for loading inorganic agents like Ag, Sr, and Zn to obtain long-term antibacterial ability and osseointegration [126, 180,181,182]. Ag nanoparticles have been incorporated into TiO2 nanotube arrays previously with satisfactory small possibility to develop resistant strains, a broad-spectrum antibacterial property, low cytotoxicity, and good stability by immersion in a silver nitrate solution followed by ultraviolet light radiation [126].张等人。 demonstrated that a series of porous TiO2 coatings with different concentrations of silver had significant inhibition effect on Escherichia coli 和金黄色葡萄球菌 . Besides, only with the optimum amount of silver can the coatings retain the antibacterial effect but without any measurable cytotoxicity to cells [183]. Due to cytotoxicity observed by the excessive release of Ag + subsequently, titania nanotube arrays with Ag2O nanoparticles embedded in the wall are prepared on Ti by TiAg magnetron sputtering and anodization in order to get slower and more controllable silver ion release [184]. That is because the TiO2 barrier is surrounded thereby minimizing the cytotoxicity induced by burst or large Ag + release.
Similar to Ag, Zn possesses antibacterial and anti-inflammation properties, and osteogenesis induction [185,186,187]. Huo et al. produced anodic TiO2 nanotube arrays at 10 V and 40 V (NT10 and NT40) incorporated with Zn by hydrothermal treatment at 200 °C for 1 and 3 h (NT10-Zn1, NT10-Zn3, NT40-Zn1, and NT40-Zn3) in Zn containing solutions, followed by annealing at 450 °C for 3 h in air. NT40-Zn3 has the largest Zn loading capacity and releases more Zn compared with other samples. The amounts of Zn released diminish gradually with time and nearly no Zn can be detected 1 month later except sample NT40-Zn3 (Fig. 11). The NT-Zn samples present different antibacterial ability. It is evident that NT40-Zn3 and NT10-Zn3 effectively kill more adherent bacteria as well as surrounding planktonic bacteria in the early stage. Figure 12a describes a synergistic effect of both released and surface incorporated Zn while Fig. 12b explains the effect of the released Zn [181].
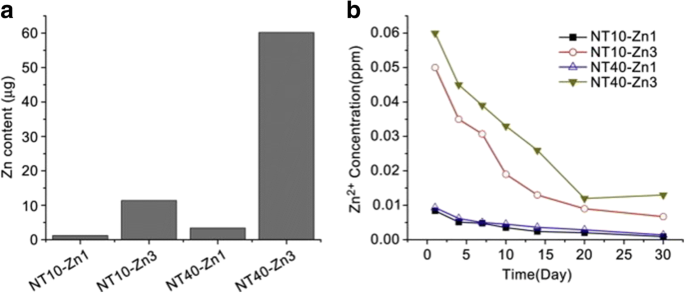
一 Total amounts of Zn incorporated into the NT-Zn samples for the 1 cm 2 coatings and b non-cumulative Zn release profiles from NT-Zn into PBS. Reproduced from ref. [181]
图>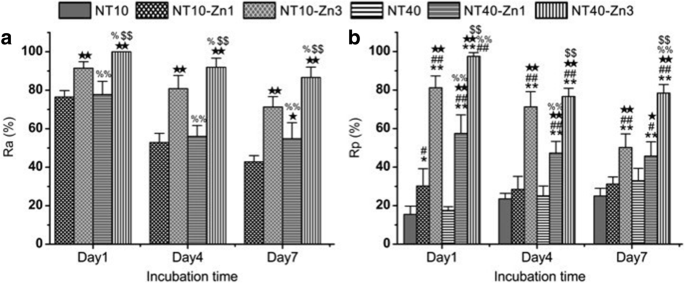
一 Antibacterial rates versus adherent bacteria on the specimen (Ra) and b antibacterial rates against planktonic bacteria in the medium (Rp) *, **p < 0.05 and 0.01 vs NT10; # , ## p < 0.05 and 0.01 vs NT40; ★ , ★★ p < 0.05 and 0.01 vs NT10-Zn1; % , %% p < 0.05 and 0.01 vs NT10-Zn3; $ , $$ p < 0.05 and 0.01 vs NT40-Zn1. Reproduced from ref. [181]
图>结论
This review presents the historical developments and traditional formation mechanism of titania nanotube arrays grown by electrochemical anodization as well as the approaches to influence and modify morphology in order to improve their performances. We also focus on current efforts toward TiO2 nanotubes applications in biomedical directions. Those steady progresses have demonstrated that TiO2 nanotubes are playing and will continue to play an important role in material science, but there are still some aspects needed to be further improved.
- 1.
The synthesis of TiO2 nanotube arrays is already comparatively mature so far in fact, but how to simplify the technology for the purpose of large-scale production in industry with extending practical operability and how to precisely control nanotube geometry efficiently by varying the anodic parameters so as to obtain optimized properties have yet to be further investigated.
- 2.
The formation mechanisms of anodic TiO2 nanotubes have gradually become a hotspot of research due to their unique structure and excellent performances but the exact mechanism remains controversial. Conventional FAD explains the growth process and the porous structure of TiO2 nanotubes, but the combination of viscous flow model and growth model of two currents can give a comprehensive explanation to the growth process. Notably, the validity of oxygen evolution resulting from electronic current has much room for investigation.
- 3.
Modification is key for improving performances of titania nanotube arrays. Thus, we need to explore more methods for modification and take full advantage of the self-organized nanostructure. Through self-assembling inorganic, organic, metallic, and magnetic nanoparticles into or onto the tubes as nanocomposites with broad spectral response to visible light, high quantum efficiency, and stabilizing properties, applications could be widened. Currently, ALD appears to be an option to coat the titania nanotube layers homogenously and precisely from the bottom to the tube mouth, resulting in many advanced functionalities of the newly prepared nanotube layers. Nevertheless, further optimization of the ALD process toward coatings and inner fillings is demanded.
- 4.
TiO2 nanotube researches in biomedical directions are still in their infancy and have a long distance to go in clinical use. The biological reaction between cells and titania nanotubes has to develop from cellular level to molecular level and from morphological changes to molecular alterations. It has been shown that adhesion, spreading, and growth of osteoblast and mesenchymal stem cells strongly depends on nanotube diameter, so the regularity and principle of this phenomenon as well as other factors affecting cells’ behaviors need to be further explored.
缩写
- ALD:
-
原子层沉积
- BMP-2:
-
Bone morphogenetic protein-2
- DMSO:
-
二甲亚砜
- DSSCs:
-
Dye-sensitized solar cells
- EBSD:
-
Electron Backscatter Diffraction
- EC:
-
Endothelial cells
- EdU:
-
A thymidine analog
- EGF:
-
Epidermal growth factor
- FAD:
-
Conventional field-assisted dissolution
- FE-SEM:
-
场发射扫描电子显微镜
- Fe-TNTs:
-
Fe-doped TiO2 nanotubes
- FIB:
-
Focused ion beam
- 开始:
-
氧化石墨烯
- GS:
-
Gentamicin sulphate
- HA:
-
Hydroxyapatite
- hASCs:
-
Adipose-derived stem cells
- IPCE:
-
Incident photon-to-electron conversion efficiency
- MSCs:
-
Mesenchymal stem cells
- NAC:
-
N -Acetyl cysteine
- N-TNT:
-
N-doped TiO2 nanotubes
- PEC:
-
Photoelectriochemical
- SBF:
-
Simulated body fluid
- 紫外线:
-
紫外线
- VSMC:
-
Vascular smooth muscle cell
纳米材料
- 铪的 4 种用途 |铪及铪合金的应用
- 用于改进诊断和治疗应用的多功能金纳米粒子:综述
- 用于合成和生物医学应用的荧光纳米材料的进展和挑战
- 用于超级电容器应用的石墨烯和聚合物复合材料:综述
- 迈向 TiO2 纳米流体——第 2 部分:应用和挑战
- 接触非平衡等离子体对 Mn Х Fe3 − X О4 尖晶石结构和磁性能的影响
- TiO2 纳米管阵列:由软硬模板制造和场发射性能的晶粒尺寸依赖性
- 天然和合成纳米材料的电化学、生物医学和热特性的比较研究
- 在阳极 TiO2 纳米管阵列上加载 CeO2 纳米颗粒的简便方法
- TiO.91O2/CdS 混合球结构光学特性的合成与研究
- 铣削主轴和卓越的应用
- 9 种穿线工具及其应用