走向 TiO2 纳米流体——第 1 部分:制备和性质
摘要
纳米流体作为新一代工作流体,在过去的三十年里一直被视为研究的热点。许多评论论文对纳米流体的发展和最新技术进行了全面系统的总结。迄今为止,由于相关文献数量庞大,对各种纳米流体进行全面综述变得越来越困难。并且在各种纳米流体中观察到了报告论点中的许多争议和不一致之处。同时,对某种纳米流体的系统或综合评价还不够。因此,本综述重点研究最热门的类型之一,即。 TiO2 纳米流体因其引人入胜的分散性、化学稳定性和无毒等有趣而全面的特性而受到科学家们的极大关注。由于纳米流体的制备是前提,物理性质是进一步应用的关键因素,本综述的第一部分总结了近年来在TiO2纳米流体的制备、稳定性和物理性质方面的研究。
评论
背景
纳米流体的开发
由于液体的传热能力通常远低于固体金属或金属化合物的传热能力,因此可以通过将固体颗粒悬浮在液体中来增强液体的传热能力。然而,毫米级或微米级颗粒的悬浮液存在一些缺点,如分散性差、聚集和沉降以及粘附在系统内表面,容易导致传热性能下降,泵送功率增加,甚至管道阻塞。当新一代悬架即。纳米流体由Choi于1995年提出[1]。
Nanofluid 是一种新型的稀释悬浮液,其中包含至少一维尺寸低于 100 nm 的纳米颗粒。当悬浮液中的粒径达到纳米级时,预计悬浮液可以获得更好的热性能,同时保持比毫米或微米颗粒/液体混合物更稳定。稳定的纳米流体也可以获得更好的流动性,有时可以将其视为单相流体。因此,纳米流体面临的最大挑战之一是制备和稳定性,这是实现良好热物理性能和进一步工程应用的主要先决条件。相应地,纳米流体的研究大体可以分为以下几个方向:制备和稳定性研究[2, 3],热导率等物理性质[4,5,6,7,8]和粘度分析[9,10] ,11,12],传热研究[13,14],工程应用[15,16,17,18],理论分析或模型开发[19,20,21,22,23,24,25]。
在过去的 20 年,尤其是最近的 10 年中,纳米流体的研究因其迷人的特性而呈爆炸式增长,许多研究人员对纳米流体的各个方面进行了实验或理论研究 [26,27,28,29]。为了说明这一点,从“科学网”检索到的标题中包含“nanofluids or nanofluid”的出版物数量的增长趋势可以在图 1 中找到。该图清楚地说明了纳米流体的研究增长如此之快,以至于2016 年的出版物占过去二十年总数的 21.9%。如果检索范围放宽到全文并包含更多的搜索数据库,结果可能会增加几倍。因此,由于相关文献数量庞大,对各种纳米流体进行全面综述变得越来越困难。在过去的 2 年里,一些评论侧重于财产的一个方面或某种纳米流体,以提供更全面的评论。例如,表 1 显示了对纳米流体某些专业方面的最新评论,例如:
- (1)
制备或表征 [30,31,32]
- (2)
某些类型的纳米粒子(Al2O3、TiO2、CuO、石墨烯、CNT、混合纳米流体)[32,33,34,35,36,37,38]
- (3)
某些类型的基液(水、EG、EG/水混合物、油)[39,40,41,42]
- (4)
一种或多种物理性质(热导率、粘度、比热)[43,44,45,46,47]
- (5)
某些特性(受迫、自然、沸腾对流传热、压降、粒子迁移)[48,49,50,51,52,53]
- (6)
一些特殊应用(热交换器、太阳能集热器、制冷)[54,55,56,57,58,59,60,61,62]
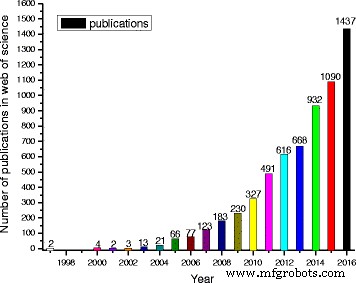
“web of science”检索标题中包含“nanofluids or nanofluid”的论文数量
图> 图>TiO2 纳米流体的优势
上述表1中的介绍体现了对纳米流体一些专业方向进行综述的可行性和意义,因为它可以提供某一方面相对全面和详细的信息。作为最流行的种类之一,TiO2 纳米流体因其优异的物理和化学性质而引起了科学家的极大关注。首先,二氧化钛广泛应用于印刷、化妆品、空气净化等领域,是公认的对人体无任何毒性的安全材料。考虑到这种纳米流体的安全性,Taghizadeh-Tabari 等人。 [63] 已将 TiO2-水纳米流体应用于牛奶巴氏杀菌行业的板式换热器中。其次,TiO2 具有优异的化学稳定性,耐酸、碱和大多数有机溶液侵蚀。第三,二氧化钛纳米粒子已经以更大的工业等级生产,这使得它们相对经济[64]。第四,TiO2 纳米粒子在极性和非极性基液中都具有较好的分散性,尤其是在添加适当的分散剂时。杨等人。 [65] 研究了 20 种纳米颗粒在氨水溶液中的分散稳定性。结果表明,锐钛矿型TiO2是不含表面活性剂的最稳定的金属氧化物,加入适当的表面活性剂可进一步提高其稳定性。在 Silambarasan 等人的报告 [66] 中,如图 2 所示,TiO2 纳米流体在储存 10 天后的吸收率变化很小。吸收率的这种微小变化表明他们制备的 TiO2 纳米流体的稳定性非常显着。总结现有文献可以得出结论,TiO2 纳米颗粒总体上比其他常规金属氧化物纳米颗粒具有更好的可分配性。由于纳米粒子在液体中的分散性是纳米流体应用的最重要前提,因此许多研究人员选择了TiO2纳米流体作为研究对象。
<图片>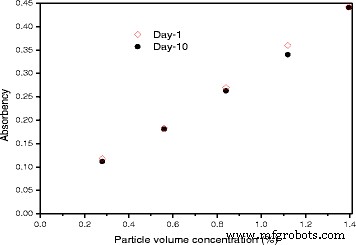
作为第 1 天和第 10 天颗粒体积浓度函数的吸光度 [66]。经爱思唯尔许可转载
图>由于纳米流体的制备是设计和构建能源相关应用的先决条件和物理性质的关键因素,这两篇综述的目的是系统地总结近年来TiO2纳米流体的研究进展,包括制备、稳定性、物理性质。和能源应用。两个关于 TiO2 纳米流体的制备、性质和应用的评论的详细示意图见图 3。这篇评论是从某种纳米流体的角度组织的,它被认为是最接近的一种到实际应用。本文的主要目的是为研究人员更新TiO2纳米流体的研究现状提供有益的参考指南,并指出未来研究方向的关键挑战和有益建议。
<图片>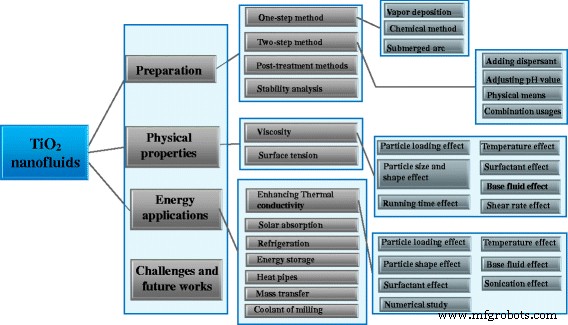
TiO2纳米流体的制备、性能、应用和挑战示意图
图>二氧化钛纳米流体的制备
一步法
通常,可以区分两种主要的制备方法:一步法和两步法。一步法是通过将纳米粒子悬浮在伴随其生成过程所需的工作流体中来实现的。一步法又可细分为物理法和化学法。物理方法包括气相沉积、激光烧蚀和埋弧。化学法是指通过化学反应产生纳米流体。一般将上述方法作为干纳米粒子的制备方法进行介绍。然而,这些方法可以通过将干颗粒收集器更换到相应的基液容器中来升级为纳米流体的一步制备方法。
气相沉积
气相沉积是制备纳米流体的常用物理方法。这种方法的典型设备可以在图 4 [67] 中查看。用于制备纳米颗粒的块状固体材料在充满惰性气体的低压容器中加热蒸发,然后原料蒸气被旋转的液膜冷却并沉淀在基液中。气相沉积通常用于制备金属纳米流体,但由于沸点较高,这种方法很少用于TiO2纳米流体。但是,可以通过使用电加热来实现高温来改进这种方法。李等人。 [68] 使用一步脉冲线蒸发 (PWE) 方法制备含有 TiO2 纳米颗粒的乙二醇 (EG) 基纳米流体。他们在一根细线上施加 25 kV 脉冲电压,并使其过热,在几毫秒内蒸发成等离子体。然后,等离子体与氩氧相互作用并凝聚成纳米颗粒。最后,他们通过让纳米颗粒直接接触腔壁内的EG获得了TiO2纳米流体。
<图片>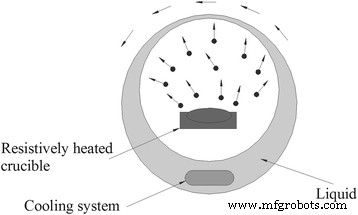
一种典型的气相沉积法制备纳米流体的装置。根据参考文献[67]重新绘制
图>埋弧法
埋弧法可以为TiO2纳米流体的制备提供并保持更高的温度。张等人。 [69] 制造了一种新的埋弧合成系统来生产 TiO2 纳米流体。他们的装置主要由电弧喷涂单元、真空空间和温度和压力控制系统组成,如图 5 所示。在该装置中,在真空中通过电弧放电方法蒸发大块 TiO2 固体,然后,气态的二氧化钛被隔离的液体迅速冷却成细小的固体。他们得出结论,这种方法比气溶胶方法更突出,因为制备的纳米流体具有更高的分散稳定性,可以被认为是牛顿流体。张等人。 [70]通过优化反应参数控制系统、冷却循环和埋弧装置的尺寸,改进了埋弧法。基于优化的系统,它们可以生产更稳定、更细的 TiO2 悬浮液,并具有良好的粒径重现性。并且其TiO2纳米颗粒的吸附性能优于商业纳米颗粒。
<图片>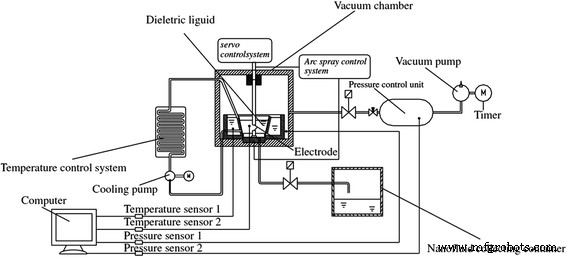
示意图改进的埋弧纳米流体合成系统 (ISANSS) [40]。经日本金属材料学会许可转载
图>化学方法
化学法是通过化学反应获得纳米流体,一般包括共沉淀法和前驱体转化法。合成 TiO2 纳米流体的常规化学方法是基于前体 TiO(OH)2 沉积物,通过无机钛盐和氨水的化学反应,然后进行煅烧以获得 TiO2 粉末。一些研究表明,通过化学方法获得的纳米流体比两步法制备的纳米流体具有更好的稳定性和更高的热导率[71]。纳米粒子微结构的可控性是该方法的另一个显着特征。常规的调节方法是控制合成温度、pH值、超声浴时间和添加剂等参数[72]。然而,该方法主要用于通过干燥液体制备TiO2粉末,由于该方法中复杂的液体环境不适合纳米流体的详细应用。而当TiO2粉末通过改变体液而无需干燥过程即可稳定地悬浮在所需的基液中时,该方法在酸碱度和电解质浓度等新液体环境参数接近原液的条件下具有前景。准备。
一步法没有包含容易形成纳米颗粒团聚的干燥和分散过程。因此,一般认为一步法可以获得更稳定的纳米流体[73]。但是,也存在一些缺陷,限制了一步法的应用范围。例如,气相沉积不能用于制备含有高沸点或非晶体纳米颗粒的纳米流体。激光烧蚀和真空埋弧方法成本高,需要苛刻的环境条件。化学方法一般需要特定反应条件的服务,如所需的pH值和温度。并且它可以很容易地在液体中合成一些副产品 [74]。例如,Sonawane 等人。 [75] 使用溶胶-凝胶法合成锐钛矿 TiO2 纳米粒子,其 pH 值恒定为 5。前体溶液包括异丙醇钛和异丙醇以及双蒸水。可以得出结论,这种具有特定pH值和复杂化学成分的混合物不能用作传热纳米流体。因此,他们将合成的 TiO2 纳米粒子干燥,然后通过超声波处理将它们重新分散到所需的基础工作流体中,包括水、EG 和石蜡油,以获得所需的纳米流体。可以得出结论,一步法几乎不适用于某些具有特定成分的纳米流体,特别是对于以纯水、油、制冷剂等为基液的纳米流体以及含有挥发性气体的应用系统。
两步法
在两步法中,生产纳米颗粒和将它们悬浮在所需基液中的过程是独立进行的。由于二氧化钛纳米粒子的合成技术已基本达到工业化生产规模,二步法广泛用于二氧化钛纳米流体。图 6 显示了两步法的典型程序。干燥的纳米粒子首先通过化学或物理方法合成,然后悬浮在所需的基液中。然而,由于颗粒间的强相互作用力可能导致纳米颗粒发生碰撞和聚集,因此它们很难稳定均匀地悬浮在基液中。因此,一般采用一些分散方法来保证纳米流体的良好稳定性和可用性。
<图片>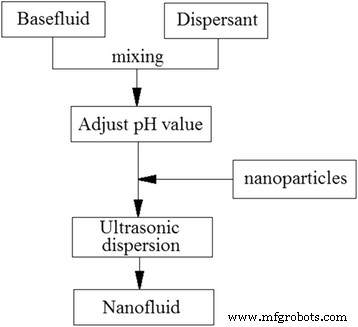
制备纳米流体的两步法的典型程序 [35]。经爱思唯尔许可转载
图>表 2 总结了近年来 TiO2 纳米流体制备方法的相关研究。可以看出,基液的种类有水、EG、制冷剂、有机溶剂等。总的来说,纳米颗粒在基液中的分散和悬浮三种主要技术在两步制备过程中得到广泛应用。
图>添加分散剂
第一种分散方法是通过添加分散剂对颗粒表面进行改性,这有望通过分散剂分子的静电排斥或空间位阻的作用来防止纳米颗粒聚集 [76]。可以注意到,现有报告中最常用的表面活性剂是 CTAB。其他种类包括SDBS、SDS、PVP、油酸、乙酸和PEG也用于一些研究。 2012 年,Mo 等人。 [77]通过将棒状金红石TiO2和球状锐钛矿TiO2悬浮在水中,采用两步法制备了两种纳米流体。他们观察到,当使用 SDS 作为分散剂时,纳米流体可以保持稳定 286 小时。第二年,他们比较了三种不同表面活性剂(包括 SDBS、PVP 和 CTAB)对分散的影响 [78]。并且他们发现在这个实验研究范围内,当SDBS和二氧化钛纳米粒子的质量比为0.3时,可以获得最佳的纳米流体分散效果。 Nakayama 和 Hayashi [79] 在丙酸和正己胺的表面改性的帮助下,使用两步法将高负载的 TiO2 纳米粒子分散在有机基液中。他们发现表面改性可以提高纳米流体的分散性,两步法的效果优于一步法。他们制备的TiO2纳米颗粒的特性没有改变,可以很好地应用于不同的有机溶剂基液。
调节 pH 值
第二种分散方法是通过调节基液的pH值来调节分散环境。该方法是通过调节流体的合适pH值来为纳米粒子配备更高的zeta电位,从而避免纳米粒子因较高的静电排斥而接触[76]。 Li 和 Sun [80] 研究了 pH 值对 SRFA 和 Fe(III) 在一元和二元基液中 TiO2 纳米粒子聚集行为的影响。他们发现 SRFA 的吸附极大地提高了 TiO2 纳米粒子在 4、6 和 8 的 pH 值下的悬浮稳定性,他们认为这主要是由于粒子表面负电荷急剧上升造成的。他等人。 [81] 发现通过将基液的 pH 值调整为 11 可以极大地提高 TiO2 纳米流体的稳定性,在该值下可以形成 45 mV 的高 zeta 电位,以防止重新团聚和沉积以及可能的后续污染铜管。具有最佳pH值的纳米流体可以保持稳定数月。此外,Vakili 等人。 [82] 和森等人。 [83] 将基液的pH值调整为11,他们发现TiO2纳米流体在这种强碱性条件下具有更好的分散稳定性。
物理方法
第三种分散方法等同于通过物理手段,例如机械搅拌、超声波和搅拌珠磨来破坏颗粒团聚。这些方法应该会产生空化振荡,从而导致剪切、断裂和分散效应 [84]。普遍认为并证明了适当的超声振动后纳米流体会更加稳定,而TiO2纳米流体的分散稳定性总结再次证明了这一点。从表 2 可以看出,几乎所有的制备过程都涉及一些物理处理。李等人。 [85] 将 TiO2 纳米颗粒分散到 MDEA 溶液中以制备 TiO2-MEDA-H2O 纳米流体。他们发现,在不添加分散剂的情况下,纳米流体可以在机械搅拌下保持稳定 48 小时。塔吉克等人。 [86] 研究了不同超声波类型(连续或不连续脉冲)对水性 TiO2 纳米流体悬浮行为的影响。结果表明,连续脉冲比不连续脉冲具有更好的破碎效果,而后者不能分离一些大的聚集体。西兰巴桑等人。 [66] 通过实验研究了搅拌珠磨和超声处理对含有亚微米二氧化钛颗粒的水基混合物的悬浮行为的影响。他们发现搅拌珠磨可以产生含有亚微米颗粒的稳定悬浮液,并且可以进一步应用超声波处理来控制 TiO2 悬浮液的传输行为。 Longo 和 Zilio [87] 比较了机械搅拌和超声波对 TiO2-水和 Al2O3-水纳米流体分散行为的影响。他们观察到,在 25 kHz 下超声处理 48 小时显示出比简单机械搅拌更好的分散效率。经过这些物理分散处理后,两种纳米流体都可以保持稳定1个月以上。
组合使用
通常,在两步法中结合添加表面活性剂、改变基液pH值和超声振动的分散方法来获得更好的纳米流体分散性能。刘等人。 [88] 将 TiO2 纳米粒子(25 nm)分散在水中以制备稳定的 TiO2 纳米流体。采用三种处理方法,包括添加 PEG1000 作为分散剂、超声振动和将 pH 值调节至 4-5 或 9-10,以获得稳定的 TiO2 纳米流体。费德勒等人。 [89]采用加入醋酸作为分散剂,根据纳米颗粒的质量分数将pH值调节至1.86~3.07的组合分散方法,并进行适当的超声处理;他们观察到纳米流体可以保持稳定至少 35 天,因为在此期间粒子的平均尺寸几乎保持不变。加迪米等人。 [90]通过加入醋酸并将pH值调节到5以及超声波振动制备了一种极其稳定的水性TiO2纳米流体。他们发现 TiO2 纳米流体在储存 1 年后仍能稳定悬浮。这三种技术的结合使用还有一些其他的例子。可以从表 2 中找到 Mo 等人。 [77, 78],Kim 等人。 [91],穆谢德等人。 [92],Jarahnejad 等人。 [93],Ghadimi 等人。 [90] 和赛义德等人。 [94] 将这三种分散技术全部利用,以达到最佳分散效果。
然而,由于在酸性和碱性条件下的腐蚀和安全性,改变基液的 pH 值将严重限制 TiO2 纳米流体作为热流体的应用范围。因此,更多的研究人员更倾向于采用其他两种分散技术,即。为实际系统中的潜在应用添加分散剂和物理手段。吴等人。 [95] 和杨等人。 [74] 打算将 TiO2 纳米流体应用于氨水吸收制冷系统。改变pH值的方法不可用,因为基液具有由氨浓度决定的特定pH范围。因此,他们采用PAA或PEG1000作为分散剂,结合超声振动来提高TiO2纳米流体的稳定性,取得了良好的效果。为了将纳米流体应用于压缩制冷系统,Peng 等人。 [96] 在 R141b 中加入 TiO2 纳米颗粒,制备出粒径分别为 25、40、60 和 100 nm 的纳米制冷剂。使用超声波处理器对纳米制冷剂进行超声处理 20 分钟。他们认为这一步对于在散装制冷剂中实现纳米颗粒的良好分散很重要。此外,他们还通过实验研究了阴离子、阳离子和非离子表面活性剂对纳米制冷剂稳定性的影响。他们观察到表面活性剂的类型是影响稳态粒度的重要因素。凯哈尼等人。 [97] 使用表面活性剂六甲基二硅氮烷和超声振动方法首先制备干燥的 TiO2 纳米粒子,然后加入蒸馏水中,超声振动(400 瓦和 24 kHz)处理 3-5 小时。他们发现制备的纳米流体可以保持稳定数天,并且没有发生沉淀。杨等人。 [98] 发现低浓度范围的表面活性剂SDBS和超声振动的使用可以改善氨水基TiO2纳米流体的悬浮行为。
后处理方法
除了传统的一步法或两步法外,还提出了一些制备纳米流体的后处理方法。一些分散较差的含有团聚纳米颗粒的原液,通过一些特殊的处理,如将团聚的纳米颗粒从原液中分解或去除,可以得到一些分散较好的纳米流体。
黄等人。 [99] 观察到搅拌器、超声波浴和超声波破碎器对改善纳米流体分散的影响是有限的。他们使用高压均质器对纳米流体进行后处理,过程如图 7 所示。在他们的研究中,颗粒的初始平均直径在经过再处理后可以减少至少一个数量级。高压均质机。并且他们发现在他们研究的所有物理分散方式中,高压均质机的效果最好。
<图片>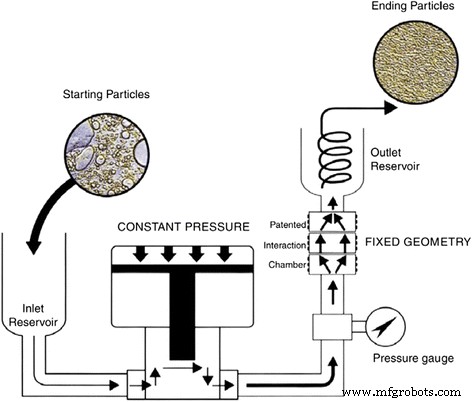
用于生产纳米流体的高压均质器示意图[99]。经爱思唯尔许可转载
图>杨等人。 [100] 使用优化方法制备纳米流体。纳米流体分散改进的优化过程如图8所示,他们从大量较高浓度的纳米流体中去除悬浮良好的纳米流体,然后通过添加基液稀释将去除的部分重新恢复到所需的浓度。如果纳米流体的吸光度与其浓度成正比,则稀释比基于特性。他们观察沉降并测量吸光度的变化以估计该方法的效果。结果表明,对于金红石型和锐钛矿型TiO2纳米流体,优化后的方法均能显着提高其分散性,制备出更稳定的TiO2纳米流体。
<图片>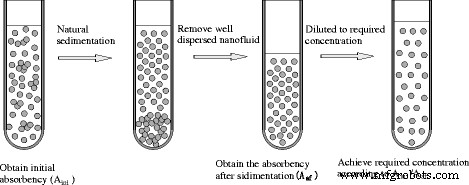
纳米流体分散改进的优化过程[132]。经 Taylor &Francis 许可转载
图>在制备纳米流体的争论中存在一些争议或不一致之处。首先,是采用一步法还是两步法是不一致的。一步法有望获得更好的分散稳定性,因为它避免了纳米粒子的干燥和分散过程。然而,一步法的副作用如副产物、特殊的溶液环境似乎更为致命,严重制约了纳米流体的应用范围。因此,由于二氧化钛纳米粒子的分散技术具有很大的适应性和实质性的改进,两步法得到了更广泛的应用。总体而言,建议采用两步法和适当的后处理方法制备TiO2纳米流体。
另一个争议是表面活性剂是否应该用于纳米流体的制备。适当的表面活性剂的存在可以提高分散稳定性,但也可能带来一些副作用,如导热系数降低、粘度增加和发泡能力。由于具有降低表面张力和提高再分散性等潜在优势,建议使用低浓度的表面活性剂,当它不会导致热导率明显下降或粘度和发泡能力增加时。此外,表面活性剂对纳米流体热导率和黏度的影响也是目前研究中存在争议的问题。
Stability of Nanofluids
Stability research is generally followed the preparation to achieve the optimal dispersion craft since it is closely related to the effectiveness and practicability of nanofluids. The great amount of aggregations in the unstable nanofluids can easily cause sedimentation and adsorption on the inner surface of the system, which will probably result in the degradation of heat transfer efficiency, raising of pumping power, and even blocking up in system pipe blocks.
It can be found from Table 2 that the stable times of different researchers thought were variously distributed in the range of several hours to 1 year. A most stable nanofluid was obtained by a combined use of adding surfactant, controlling pH value, and ultrasonic vibration by Ghadimi et al. [90]. Also, the particles’ loading in their experiment was very low at 0.1 wt.%, which was also another contribution for the long-term stability. Without adding surfactant, the nanofluids can also achieve a better dispersion stability by adjusting the pH value of the liquid to a suitable value. For example, He et al. [81] and Longo et al. [87] observed that the TiO2 nanofluids can keep stable for months by adjusting the pH to 11 with the help of ultrasonic vibration. Also, some TiO2 nanofluids with good dispersion stability were prepared only through physical means in some research. Padmanabhan et al. [101] used a magnetic stirring to prepare R134a and mineral oil-based TiO2 nanofluids that can keep stable for 6 months. This is likely because the particles’ loading employed in their study is very low (0.1 g/L) and the high viscosity of the R134a and mineral oil base fluid can also provide a superior dispersion condition. This conclusion can also serve as proved by Palabiyik et al.’s results [102]. They obtained a TiO2 nanofluids stable for several months by the help of sonication with a higher viscosity propylene glycol as base fluid. The similarity is that they were both using organic solvent of high viscosity as base fluids and the best ones was only treated by physical means. Also, it can be seen that TiO2 nanoparticles have a comprehensive dispersivity in both polar aqueous solution and nonpolar organic solution.
However, the above judgments on dispersion stability of various TiO2 nanofluids are not very objective and accurate because most of the results showed the least stable time. Moreover, there is no uniform standard for evaluating the stability of nanofluids, and the stability evaluating methods in different research were sufficiently different. Current evaluation methods of stability of nanofluids mostly consisted of observing the stratification or sedimentation and testing the zeta potential, particles’ size, or absorbency. Mansel et al. [103] used the sedimentation observation method and zeta potential method to evaluate the stability of TiO2–water nanofluids in different pH values. They observed that in low or high pH value, the TiO2–water nanofluids can obtain good stability. Mo et al. [78] used zeta potential method to investigate the stability of TiO2–water nanofluids with three different surfactants SDBS, PVP, and CTAB, respectively. By comparing the value of zeta potential, they obtained the optimal kind of surfactant and the best dispersion of nanofluid. Wei et al. [104] used sedimentation, zeta potential (ζ), and size analysis to evaluate the stability of diathermic oil-based TiO2 nanofluids. They found that there was not obvious sedimentation and the zeta potential (ζ) and size analysis also showed good results. They thought the TiO2 nanofluids they prepared were very stable and can be used to enhance heat transfer for a fluid system.李等人。 [105] used sedimentation observation to investigate the stability of TiO2–MDEA–water nanofluids. They found that after a specific period of mechanical agitation, the sedimentation was reduced and the stability of nanofluids was improved. However, the ultrasonic vibration will deteriorate the stability of TiO2–MDEA–water nanofluids. For this reason, only mechanical agitation was employed in their research.杨等人。 [74] investigated the dispersion behavior of 20 types of nanoparticles in binary base fluid of ammonia–water by measuring the absorbency of nanofluids, and they defined ratio of varying absorbency to quantitatively compare the suspending stability of different kinds of nanoparticles, dispersant, and base fluid mixtures. They observed that the new defined index was more applicable than conventional means because it could directly compare the suspending behavior of various kinds of nanofluids. While the method of observing the stratification or sedimentation is restricted for nanofluids in different colors or without distinctly stratification after standing. The results showed that the anatase and rutile TiO2 nanofluid were the most stable metal oxides without any surfactant. And when adding optimal dispersant, anatase TiO2 nanofluid was still the most stable one.
Generally, the combination of several stability evaluating methods is employed to investigate the stability of nanofluids more accurately. Silambarasan et al. [66] used method of measuring the particle size distribution, zeta potential, and microscopy of grain size methods to characterize the suspending stability of TiO2 nanofluids. By those methods, they prepared remarkably stable TiO2 nanofluids whose absorbency changed very little after 10 days. Tajik et al. [86] used sedimentation observation and microscopy of grain size to investigate the roles of ultrasonic wave types on the suspending behavior of nanofluids. And they found that the pulses in discontinues type could not smash some big clusters or aggregations since the sedimentation occurred after 48 h of storage.
Physical Properties of TiO2 Nanofluids
The physical properties of TiO2 nanofluids are focused on the viscosity and thermal conductivity. Also, a few papers investigated the surface tension. Using nanofluids to enhance the thermal conductivity is a typical application in heat transfer filed. Therefore, the thermal conductivity of TiO2 nanofluids will be introduced in part 2 of the reviews. In part 1, the viscosity and surface tension are introduced as follows.
Viscosity
Viscosity is an essential parameter for nanofluids especially for flow and heat transfer applications because both the pressure drop and the resulting pumping power are depended on the viscosity. Viscosity describes the internal resistance of a fluid to flow, and it is an important property for all thermal and flow applications for nanofluids. The nanofluids with higher viscosity will result in higher flow resistance and lower flow velocity, which also induce the decrease of the heat transfer. To obtain flow velocity and heat transfer efficiency, more pumping powers are needed which induce more energy consumption. Moreover, for some mass transfer application of nanofluids, viscosity plays more important roles than thermal conductivity because the viscosity determines the mass transfer resistance of molecules entering the liquid surface and the diffusion coefficient in the liquid. Murshed and Estellé [106] provide a state-of-the-art review on the viscosity of various nanofluids. They found that the experimental data from various literatures are greatly scattered and not consistent even for the same nanofluids. This review will discuss in detail the influence factors on the viscosity of TiO2 nanofluids to provide an exhaustive knowledge on this topic.
Particle Loading Effect
Many literatures have concerned the volume concentration effect on the viscosity of TiO2 nanofluids. Table 3 shows the particle loading dependence of the viscosity of TiO2 nanofluids in different research. It can be observed that the viscosity of the TiO2 nanofluids increases with the increase of the particle loading. However, some works showed that the viscosity ratio varies linearly with variation of volume concentration, but some other results showed the viscosity ratio variation is parabolic. The viscosity enhancements of TiO2 nanofluids were greatly distinguishing in various researches. For example, in Vakili et al. [82], Arulprakasajothi et al. [107], Duangthongsuk and Wongwises [108], Saleh et al. [109], and Mahbubul et al.’s [110] results, the increments of viscosity were below ten times of the volume percentage of the added particles. However, He et al. [111] and Turgut et al.’s [112] results showed that the viscosities were increased by more than 100 times of the volume percentage of the TiO2 particles added. There are also many results distributed between the values in the above two extreme cases. Therefore, it can be concluded that the influence of particle loading on the viscosity of TiO2 nanofluids is more complex than that on thermal conductivity due to the widespread data in various studies.
图>Temperature Effect
Besides the volume concentration effect, the temperature effect on the viscosity of TiO2 nanofluids is also widely studied by many researchers.他等人。 [111] prepared four different concentration TiO2–H2O nanofluids with 20 nm TiO2 and measured the viscosities of TiO2–H2O nanofluids and deionized water with different temperatures. They observed that the TiO2–H2O nanofluids were Newtonian fluids, which were the same as Chang and Liu’s finding [69], and the viscosities varied inversely with the temperature of the TiO2–H2O mixture system. Ling et al. [113] also measured the viscosities of the TiO2–H2O nanofluids with different mass fractions, when temperature varied from 15 to 40 °C. They found that the viscosity of the nanofluids increased when fluids thicken and decreased with the increment of the temperature exponentially.刘等人。 [114] figured that the viscosities of TiO2–H2O nanofluids increase remarkably with the volume fraction of nanoparticles and vary oppositely to the temperature of the TiO2–H2O nanofluids greatly with similar experimental method. Based on the value of the viscosities, they also propose an amended suspension viscosity formula. Some research results showed that the viscosity of nanofluids is a function of volume loading and temperature as well as base fluid viscosity. Yiamsawas et al. [115] measured the viscosity of TiO2–water with a volume loading varied from 1 to 8% at a high-temperature range of 15 to 60 °C. By comparisons with previous studies, they proposed a useful correlation for practical applications which indicated that the viscosity of nanofluids is a function of volume loading and temperature as well as the base fluid’s viscosity.
Comparing with the absolute viscosity, the varieties of relative viscosity at different temperatures were more impressive for researchers. Jarahnejad et al. [93] carried out a detailed study on the effect of temperature on the viscosity and the relative viscosity of TiO2 respectively. And the results are shown in Fig. 9. It can be found that compared to base water, the average viscosities of TiO2 nanofluids increased by 17, 50, and 78% for 3, 6, and 9 wt.% of particles’ loading, respectively, at 20 °C. The viscosity of nanofluids with different particle loading decreased as the temperature increased, while the relative viscosity remained nearly constant with the temperature. The observation of independent of temperature can be also included in some other research. Fedele et al. [89] presented the characterization of water-based nanofluids where TiO2 ranging between 1 and 35% in mass. They concluded that the relative viscosity was independent from temperature for all the particle loading employed. And the nanofluids at 1 wt.% exhibited a water-like behavior within the experimental error. But this observation was invalid at the higher concentrations (+243% for 35 wt.% at 343 K). Also, Silambarasan et al. [66] found that the temperature has a smaller effect on the relative viscosity since the viscosity of TiO2 suspensions was reproducible even after repeated and alternating heating and cooling processes. And they attributed the reason to the effect of particles’ temperature-dependent intermolecular forces in the suspension. However, some different results can also be observed. Teng et al. [116] found that the relative viscosity increased from 8.2 to 16% when the temperature varied from 10 to 40 °C for the TiO2 nanofluids with 0.5 wt.% of particle loading. Cieśliński et al. [117] found that the relative viscosity of thermal oil-based TiO2 nanofluids remained constant when the temperature varied from 20 to 40 °C, but had a nearly linear increase with the increase of temperature when exceeding 40 °C. Yapici et al. [118] observed that the effect temperature was different for different shear rate. The relative viscosity measured was independent of the temperature at a higher shear rate region. However, for lower shear rate region, a great temperature dependency behavior of viscosity of TiO2 nanofluids was exhibited especially at high temperatures
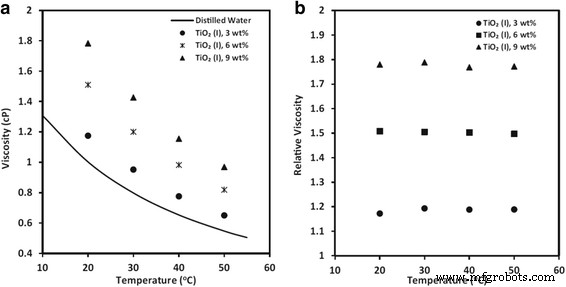
Dynamic viscosity (a ) and relative viscosity (b ) for TiO2 water-based nanofluids at different temperatures [93]. Reproduced with permission from Springer
图>Particle Size and Shape Effect
The particle size and shape effects on the viscosity of TiO2 nanofluids were not investigated as widely as that of particles’ loading or temperature. In particular, Chen et al. [64, 119] investigated experimentally the viscosity of spherical (25 nm) and rod-like (10 × 100) TiO2 nanoparticle-based nanofluids with water and EG as base fluid, respectively. They found that the viscosity of TiO2 nanofluids was more sensitive to the rod-like particles than spherical particles. It can be seen from Table 3 that the viscosity was increased by 0.5–23% when adding 0.1–1.86 vol.% of spherical TiO2 nanoparticles, while increased by 1–82% when adding 0.1–0.6 vol.% of rod-like TiO2 nanoparticles. The same observation can also be found for EG-based nanofluids.
Surfactant Effect
The surfactants have been observed to have great effects on the viscosity of TiO2 nanofluids in some recent research. Jarahnejad et al. [93] investigated the effect of two kinds of surfactant trioxadecane acid and poly carboxylate on the viscosity of TiO2 nanofluids respectively. Their results of the dynamic viscosity of 9 wt.% TiO2–water nanofluids with different surfactants vs. temperature are shown in Fig. 10. The results demonstrated only a very slight increase was found in the viscosity of nanofluids even with the highest particle loading viz. 9 wt.%. However, the two kinds of surfactants could greatly increase the viscosity of nanofluids in the temperature range of 20–50 °C, especially for trioxadecane acid. The similar effect of surfactant on viscosity can also be observed in Ghadimi and Metselaar’s report [90], in which they found SDS can also increase the viscosity of TiO2 nanofluids with 0.1 wt.% particle loading. It was also observed there were important roles of SDS in the long-term dispersion stability of TiO2 nanofluids. Therefore, they still suggested that the dispersion method of adding surfactant and ultrasonic vibration to be adopted in the preparation of nanofluids.
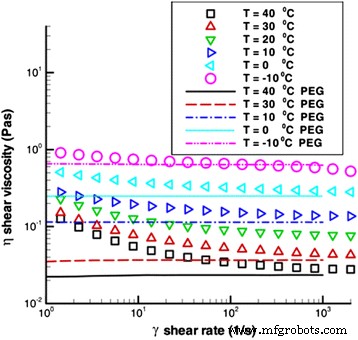
Dynamic viscosity of 9 wt.% TiO2–water nanofluids with different surfactants vs. temperature [93]. Reproduced with permission from Springer
图>However, the above results cannot prove that all kinds of surfactant will result in high viscosity for nanofluids. Figure 11 shows the viscosity of TiO2 nanofluids with PEG600 as surfactant measured by Bobbo et al. [120]. It can be seen that the viscosity of base water will not increase but decrease slightly when adding PEG600 at 0.02 or 0.2% loadings. Also, the viscosity of nanofluid containing 0.01% TiO2 nanoparticles and 0.02% PEG600 was a little lower than that of the base water. However, for higher loading of PEG, the viscosity will be greatly increased whether or not containing nanoparticles. It can be seen from Fig. 11 that the nanofluids containing 2% PEG600 and 1% TiO2 nanoparticles showed a viscosity higher than 7% in respect to water, which was analogous at each temperature. The above observation showed the viscosity of nanofluids can be lower than the base fluid in some cases, which also occurred in SWCNT nanofluids in their experiment. The decline of viscosity of fluid when adding surfactant or nanoparticles was also been found in some other research.杨等人。 [121] found that emulsifier OP-10 can reduce the viscosity of ammonia–water in lower concentrations. Ling et al. [122] observed that adding SDBS or OP-10 in TiO2 nanofluids with a lower loading can induce a slight drop in viscosity. Therefore, it is an important issue to choose the suitable surfactants to improve the dispersion stability without increasing the viscosity significantly.
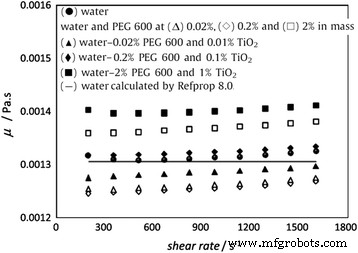
The viscosity of TiO2 nanofluids with PEG600 as surfactant [120]. Reproduced with permission from Elsevier
图>Base Fluid Effect
The information about base fluid effect on viscosity can be illuminated though Chen et al.’s study [119], in which they found the relative increments of viscosity of water-based TiO2 nanofluids were distinctly higher than that of EG based. It seemed that the higher viscosity the base fluid could result in lower increment in viscosity. Mahbubul et al. [110] found that the viscosity of R123 was increased by only 5.2% when adding 2 vol.% TiO2 nanoparticles. Sen et al. [78] and Yapici et al. [118] found relative increments of viscosity about 20 times of the particles’ volume percentages. It also seems that TiO2 nanoparticles are more suitable in the organic liquid because a lower relative increment in viscosity can be obtained especially at the higher temperature. Yiamsawas et al. [123] conducted experiments on a mixture with TiO2 nanoparticles and EG/water (20/80 wt.%) in which the volume loading ranged from 0 to 4% and temperature ranged from 15 and 60 °C. They used the experimental data to present a useful correlation to predict the viscosity.
Shear Rate Effect
Another main distinction on the viscosity of TiO2 nanofluids in different research is that whether the fluids were Newtonian fluids in different shear rates. A typical Newtonian nanofluid can be found in foregoing Fig. 11. However, it can be observed from Table 3 that more than half of the results showed that the TiO2 nanofluids in their work are Newtonian fluids, but some others come to the opposite conclusion. Research on rheological characteristic has demonstrated that whether or not the TiO2 nanofluids exhibit Newtonian behavior is also affected by other factors, including the base fluid type, temperature, and particle loading. A quintessential example can be found in Chen et al.’s research [64], where they measured the viscosity of four types of nanofluids made of TiO2 nanoparticles (25 nm) and TiO2 nanotubes (10 nm × 100 nm) dispersed in water and EG. They found that EG–TiO2 nanofluids exhibited Newtonian behavior, whereas water–TiO2, water–TNT, and EG–TNT nanofluids exhibited non-Newtonian behavior. They indicated that the rheology behavior of TiO2 nanofluids is affected by their specific ingredient and environment, such as particles’ shape and liquid circumstance. The rheological characteristic of TiO2 nanofluids is also related to the temperature. Yapici et al. [118] investigated the rheological characteristic of 9 wt.% TiO2–water nanofluids with different surfactants vs. temperature. The results are shown in Fig. 12. It can be observed that the base fluid PEG was a typical Newtonian fluid in all kinds of temperature. However, TiO2–PEG200 nanofluids were nearly Newtonian fluid at a lower temperature and higher shear rate, but it changed into non-Newtonian fluid at higher temperature and lower shear rates. Also, in Said et al.’s results [94], the TiO2 nanofluid with 0.1 vol.% loading was Newtonian fluid at 55 °C, whereas it was non-Newtonian below this temperature for 0.3 vol.% particle loading.
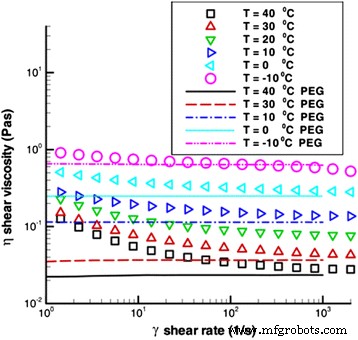
Shear rate dependency of viscosity as a function of temperature for 5 wt.% TiO2–PEG200 nanofluids [118]. Reproduced with permission from Springer
图>Running Time Effect
When the nanofluids are actually used in a running system, the time-dependent properties of nanofluids should be a crucial issue for the sustainable application. However, this matter has not been widely studied because of the faultiness in the development of nanofluids. It is generally considered that the thermal and rheological properties of nanofluids will be deteriorated due to the aggregation of nanoparticles after running a long time in the system. However, an opposite result in the time-dependent viscosity of TiO2 nanofluids can be observed in Said et al.’s research [94]. Their results for viscosity of TiO2–water nanofluid with different volume loading and temperature as well as running time are shown in Fig. 13. It can be observed that the viscosity of fresh samples and the stale samples after running in a flat plate solar collector for 1 month were distinctly different. The viscosity of TiO2 nanofluids was decreased after undergoing the alternative variations in temperature and flow rate in the cycle. This observation was quite interesting and could not be explained anywhere else in the literature. They thought this finding could open new research scope for the applications of nanofluids for a long-term use.
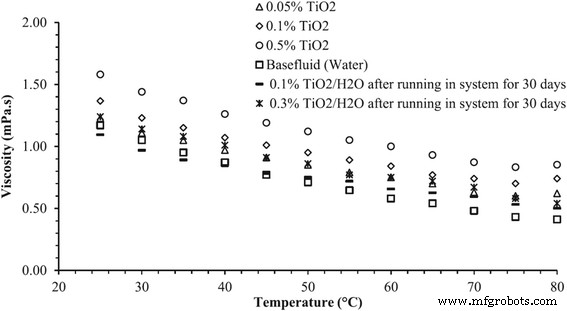
Viscosity of TiO2–water nanofluid with different volume concentrations and different temperatures [94]. Reproduced with permission from Elsevier
图>An inconsistency in viscosity of TiO2 nanofluids is quite evident. The intensities of growth in viscosity of TiO2 nanofluids with particle loadings greatly differ in various studies. And there is not yet a universal agreement on the effect of temperature, base fluid, and surfactant on viscosity of TiO2 nanofluids. Moreover, the biggest controversy on viscosity of nanofluid is that whether nanofluid is Newtonian fluid or not. The results in Table 3 exhibit that a substantial part of TiO2 nanofluids in their work are Newtonian fluids, but also, some others exhibit non-Newtonian behavior. The pronounced differences in different samples are mainly due to the complex influence factors on the rheological property. The shear rate has been proved to have great effect on the rheological property, and also, it has combined effect with other factors including temperature, shearing time, particle loading, base fluid type, and particle shape [124], which make it rather difficult to predict whether a nanofluid is Newtonian fluid or not except by experimental means.
Surface Tension of Nanofluids
The research on surface tension of TiO2–H2O nanofluids is much less than that of thermal conductivity or viscosity. Some results showed that adding TiO2 nanoparticles had little effect on the surface tension of nanofluids.刘等人。 [125] prepared TiO2–H2O nanofluids whose particle size ranged from 11 to 50 nm and the surface tensions TiO2–H2O nanofluids were investigated experimentally. They found the surface tension had no obvious change with the increase in particle loading because the surface tension of nanofluids (1% mass fraction) increased only 1.6% compared with deionized water. Hu et al. [126] found the surface tension of TiO2–H2O nanofluids increases slightly when adding nanoparticles. And the surface tension decreased as an increase in temperature. Buschmann and Franzke [127] found that no obvious variation occurs when adding a high-volume fraction (5 vol.%) of TiO2 nanoparticles in water. Tian and Wang [128] measured the surface tension of TiO2–water nanofluids by Jolly balance and abruption method. They found that the surface tension behavior of TiO2–water nanofluid was the same as water viz. the surface tension decreased as the temperature increases. However, the variation of surface tension is related to the content of nanoparticles. When the content of nanoparticles increases rapidly, the decrease rate of surface tension of TiO2–water nanofluids will slow down.杨等人。 [129] observed that nanoparticles have little effect but the surfactant can greatly change the surface tension of nanofluids, when the loading of surfactant is below the critical micelle concentration (CMC). And they explained this appearance as follows:The effect of surfactant on the surface tension of liquid is much greater than that of nanoparticles. When adding nanoparticles into a fluid containing surfactant whose loading is below CMC, the “free” surfactant will be absorbed on the surface of nanoparticles and then immersed in the liquid, which can weaken the reducing effect of surfactant on the surface tension of liquids.
However, some results also revealed that the nanoparticles played an indispensable role in the surface tension of nanofluids. Chinnam et al. [130] measured the surface tensions of Al2O3, ZnO, TiO2, and SiO2 nanofluids with a mixture of 60% propylene glycol and 40% water as base fluids, respectively. They only used one average particle size of 15 nm for TiO2 nanofluid due to limiting of manufacturer. They presented a single correlation as a function of volume loading and particle size as well as temperature for all the nanofluids by statistical analysis based on the experimental results. The experimental and fitting results related to TiO2 nanofluids are shown in Fig. 14. It was observed that the surface tension of nanofluids decreased as the temperature and particle volume loading increase and the correlation perfectly fitted the experimental data. In addition, they also observed that the surface tension decreased as the particle size decrease for a certain loading and temperature of nanofluids except the ZnO nanofluid.
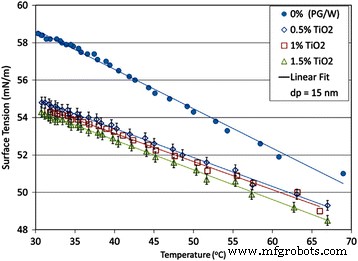
Variation of measured surface tension values of the TiO2 nanofluids with temperature [130]. For different volumetric concentrations up to 1.5% and containing 15 nm particles. Reproduced with permission from Elsevier
图>Although the surface tension study of nanofluid is not as prevalent as studies in thermal conductivity or viscosity, surface tension is also an important parameter which can affect the film flow especially the initial infiltration of film and the probability of forming channel flow. Due to the effect of surfactant on surface tension of nanofluids is greater than nanoparticles, some researchers thought that the reduction in surface tension by surfactant SDBS can produce a superior enhancement of pool boiling performance in R141b-based nano-refrigerant [131].
Conclusions
The first part of the review focuses on the preparation and two properties viz. viscosity and surface tension of TiO2 nanofluids. It can be concluded that although one-step method is expected to achieve better dispersion stability, the side effects of the one-step method such as producing by-product and requiring special solution environment seem more fatal because they severely restrict the application scope of nanofluids. Suitable treatments such as adding dispersant, adjusting pH values, and physical means (stirring and sonication) used singly or in combination can greatly improve the dispersion stability. And the two-step method is recommended to be employed with appropriate post-treatment for the preparation of TiO2 nanofluids.
Particle loading is positively correlated to the viscosity, but the effects of other factors are not unified. The viscosities greatly differ in different researches which make the viscosity models hard to predict the experimental value, and hence, the experimental mean is firstly recommended. The surface tension of TiO2 nanofluids is more sensitive to surfactant than nanoparticles. The surfactant with low concentration is suggested to be used when it not brings obvious increase in viscosity and foaming ability due to the potential advantages such as reduction in surface tension and improvement in re-dispersible property.
纳米材料