纳米通道控制合成超高氮掺杂效率的介孔 Fe/N/C 催化剂用于氧还原反应
摘要
设计适当的方法以有效提高氮掺杂效率和活性位点密度对于提高非铂 Fe/N/C 型电催化剂的氧还原反应 (ORR) 活性至关重要。在这里,我们提出了一种简单有效的策略,通过分子筛限制的 Fe 2+ 离子与 2,4,6-tri(2-pyridyl)-1,3,5-triazine 络合物配位作为具有稳定配位效应的新型前驱体。将纳米通道限制效应与稳定的配位效应相结合,可以协同提高热稳定性和稳定富氮活性位点,有助于控制热解过程中活性氮原子的损失,进一步获得高活性位点密度。增强 ORR 活性。所制备的 Fe/N/C 电催化剂表现出优异的催化活性,起始电位约为 0.841 V(相对于 RHE),与 Pt/C 催化剂非常接近,并且在碱性电解质中具有较高的长期稳定性。此外,该催化剂的过氧化氢产率低(<6.5%)和电子转移数高(3.88-3.94),表明它是传统 Pt/C 催化剂的有价值的替代品。该工作为设计高性能Fe/N/C电催化剂开辟了新途径,加深了对活性位点和ORR催化机理的理解。
介绍
探索先进的清洁能源装置(如燃料电池和金属空气电池)是当前解决环境污染和能源危机的有效途径。在这些系统中,具有缓慢动力学和反应途径多样性特征的氧还原反应(ORR)是一个关键过程[1, 2]。目前,ORR的一流和广泛使用的电催化剂是锚定在各种碳材料上的铂纳米粒子,但它们存在成本高、稀缺、稳定性差、易中毒等缺点,在很大程度上限制了大规模商业应用[3]。因此,设计廉价、高效的非铂或非贵金属ORR电催化剂是必不可少的。
近年来,掺杂碳电催化剂因其低成本、高性能、耐腐蚀和资源丰富等优点而被认为是潜在的非贵重电催化剂之一,可替代商业铂基催化剂。 [4, 5]。过渡金属/氮掺杂碳 (TM/N/C) 作为最重要的掺杂碳 ORR 催化剂已成为一个受欢迎的研究领域 [4,5,6]。一方面,由酞菁、卟啉及其含有 TM-Nx 的衍生物衍生的非铂 TM/N/C 催化剂的合成 (x =2, 4, 6, et al.) 结构作为有前途的前体 [4,5,6,7,8,9,10],但关注的领域通常仅限于 TM-N 的结构 <我> x 是成功设计 TM/N/C 催化剂的唯一先决条件。另一方面,一些研究人员提出金属-大环配合物的高温热处理可以部分或完全破坏原来的TM-Nx 结构,然后有效的活性位点结构将被再生[11]。发现包含 TM-Nx 前体中的配位结构不是必要条件,几乎含有 TM、N 和 C 源的前体可用于 TM/N/C 催化剂[12]。这一突破促进了各种 TM/N/C 催化剂的合理构建和性能控制,特别是 Fe/N/C 催化剂作为替代商业 Pt 基催化剂的最理想产品 [13,14,15]。然而,由于 Fe/N/C 催化剂的异质结构,ORR 活性位点的识别仍然存在争议。关于Fe/N/C催化剂的ORR活性位点结构有两种观点:(i)新型Fe-Nx 在高温下对三元前驱体进行热处理后,活性结构由 Fe 和 N 原子重整 [2, 7, 10, 13, 14]; (ii) 在热解过程中由氮原子修饰的碳载体衍生的 N 掺杂碳 (NC) 结构,但 Fe 原子被认为是促进 NC 结构形成的促进剂,并且它们在热解过程中几乎没有催化作用或没有催化作用ORR 过程 [16,17,18]。尽管Fe原子在Fe/N/C催化剂中的作用尚未得到明确解释,但将N原子的掺杂引入碳载体的碳骨架中以提高电催化活性是明确的。
一个令人担忧的问题是,通过直接碳化大分子聚合物 [12, 19, 20]、化学复合物和含有 Fe-Nx 的生物蛋白质制备 Fe/N/C 催化剂 结构 [15, 21, 22] 和其他铁氮源 [17, 23, 24] 通常在开放系统中进行。此外,大多数富氮前驱体在碳化过程中容易发生聚集或团聚,这会阻碍催化ORR活性位点的有效暴露,促进N原子的热损失,降低活性位点密度,导致Fe/N/C 催化剂的 ORR 性能的限制 [25]。另一个问题是合成的 Fe/N/C 材料的电子电导率也决定了它们的 ORR 活性。一般来说,当Fe/N/C催化剂获得高电导率时,活性氮原子在煅烧过程中会严重流失。换句话说,在开放系统中不能同时考虑电导和活性位点数。控制热解过程中活性氮原子的损失仍然是提高电催化活性的紧迫问题。几个研究小组提出了一些有效的限制反应策略来改善这种现象。一些典型的样品如下:(i)具有~1 nm受限层间空间的蒙脱石用作二维空间受限反应器,以避免活性氮原子的堆积和热损失并增加电导特性[26] ,(i)过饱和氯化钠作为全封闭反应器出现,以控制氮损失并增加活性位点密度 [27],以及(iii)我们小组最近使用自组装 3D-NaCl 聚集体作为半封闭限制反应器有效降低活性富氮结构的分解速度,导致活性位点密度增加和ORR活性增强[25]。然而,这些方法通常需要繁琐的预处理、复杂的工艺或对超高温的抵抗力有限。
在这里,我们提出了一种简便易行的策略,通过分子筛限制的 Fe 与 2,4,6-三(2-吡啶基)-1,3,5- 协调的纳米通道设计新的 Fe/N/C 催化剂三嗪配合物(Fe-TPTZ)作为一种具有稳定配位效应的新型前驱体。在高温碳化过程中,这种受限方法可以减少N原子的部分损失,改善BET表面积和介孔特性,提高氮掺杂效率和活性位点密度,增加电子电导率,可以有效地促进Fe/N/C催化剂的ORR电催化活性。进一步研究了Fe/N/C催化剂的ORR动力学行为和催化机理。在 Fe/N/C 催化剂上可以观察到优异的 ORR 活性,起始电位为~0.841 V(相对于 RHE),非常接近 Pt/C 催化剂,并且具有高长期稳定性,表明它是传统的有价值的替代品。碱性溶液中的 Pt/C 催化剂。该研究的未来影响为通过整合稳定的分子级配位效应和纳米通道限制效应来设计高性能非贵重Fe/N/C催化剂提供了新的思路或方法,并加深了对活性位点的理解和理解。 ORR催化机理。
方法
介孔 Fe/N/C 催化剂的合成
为合成Fe/N/C催化剂前驱体,0.2 g KIT-6分子筛(KIT-MS)(江舒吉昌纳米科技有限公司提供)BET表面积为780m 2 g -1 超声分散在0.2 mol l -1 HCl 溶液(总体积 20 ml)。随后,依次加入0.2 g上海阿拉丁生化技术有限公司提供的2,4,6-三(2-吡啶基)-1,3,5-三嗪(TPTZ)和0.0423 g FeCl2·4H2O基于固定条件:n (Fe 2+ ):n (TPTZ) =1:3 并在 25 °C 下进一步搅拌 5 h 以充分保证 Fe 2+ 之间的配位反应 离子和 TPTZ 并有效地将形成的 Fe-TPTZ 复合物固定到 KIT-MS 的纳米通道(孔径 4-10 nm)中。在干燥箱中在 80 °C 下干燥后,所得样品标记为 Fe-TPTZ@KIT-MS 前驱体,在不同温度(800 °C、900 °C 和 950 °C)下进一步加热在 N2 流速为 0.5 L min -1 的管式电阻炉中加热 2 h .所得样品经氢氟酸(40 wt.%)完全蚀刻,再用重蒸馏水反复洗涤,得到三种介孔Fe/N/C催化剂(标记为m -Fe/N/C-800, m -Fe/N/C-900 和 m -Fe/N/C-950,分别)。具有高活性位点密度的介孔 Fe/N/C 催化剂的设计过程如图 1 所示。值得注意的是,KIT-MS 可以被视为一种新的纳米通道限制反应器,用于有效控制热分解损失氮原子并进一步提高高温热解过程中氮掺杂的活性位点密度。此外,Fe-TPTZ配合物作为制备具有高活性位点密度的介孔结构的Fe/N/C电催化剂的重要原料的主要原因是Fe 2+ TPTZ配体中的离子和氮原子,可以热稳定Fe-TPTZ复合结构并促进含Fe物种在最终Fe/N/C催化剂中的分散。同时,它可以有效降低热解过程中氮原子的热分解速度,提高氮掺杂催化活性位点密度,从而优化 ORR 性能。作为对照,我们准备了 m -Ni/N/C-900 和 m -Cu/N/C-900 通过在 900 °C 下使用相同的合成方法并使用 KIT-MS 作为新的纳米通道限制反应器。我们还在相同的热处理温度下合成了 Fe/N/C-900 和 N/C-900 催化剂,分别衍生自 Fe-TPTZ 配合物和 TPTZ 配体,而不使用 KIT-MS 作为纳米通道限制反应器.
<图片>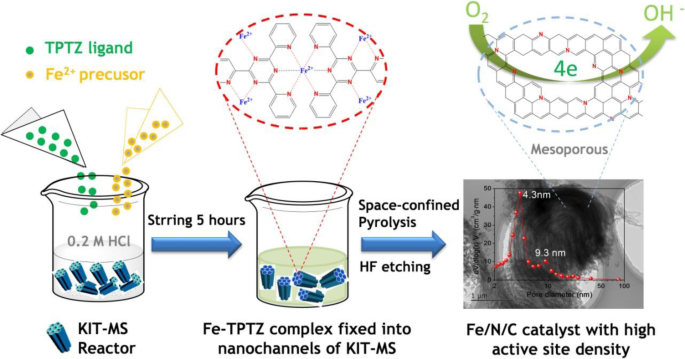
介孔Fe/N/C催化剂合成示意图
图>介孔 Fe/N/C 催化剂的表征
热重分析数据在Shimadzu DTG-60H差热分析仪上在氮气气氛下以10 °C min -1 的加热速度获得 . X 射线光电子能谱 (XPS) 数据在 Kratos XSAM800 光谱仪上收集。分别在 Hitachi UHR S4800 和 FEI Tecnai-G2 F30 仪器上获得高分辨率扫描和透射电子显微镜图像。氮吸附/解吸等温线在 Micromeritics ASAP 2010 分析仪上在 77 K 下测试。X 射线衍射 (XRD) 数据在 Shimadzu XRD-6000 X 射线衍射仪(Cu Ka1 辐射,λ)上获得 =1.54178 Ǻ) 扫描速率为 4° min -1 .使用Horiba HR800拉曼系统测量拉曼光谱,激发波长为532 nm。
电化学测试
所有电化学数据均在具有三电极系统的 CHI760E 双恒电位仪(上海晨华仪器有限公司,中国)上收集,该系统由旋转环(Pt)-圆盘(玻璃碳,Φ =5 mm) 工作电极 (RRDE, American Pine Instrument Co., Ltd.)、饱和甘汞参比电极 (SCE) 和 Pt 箔对电极 (1 cm 2 )。催化剂涂层 RRDE 的制备参考之前的报道 [21, 25]。通常,10 μl 10 mg ml -1 将催化剂分散体滴在 RRDE 上并自然干燥。催化剂的质量负载限制在约600 μg cm -2 .根据能斯特方程,碱性电解质中所有电极电位与 SCE 的关系都被转换为可逆氢电极 (RHE) 的电位。换句话说,电位转换遵循以下等式:E (vs. RHE)/V =E (vs. SCE)/V + 1.0 V。在进行电化学测试之前,催化剂涂覆的 RRDE 的活化是通过以下方式进行的:循环伏安测试从 1.2 到 0.2 V vs. RHE 在 0.1 mol l -1 用氮气饱和 KOH 溶液 20 个循环。扫描速率为5 mV s -1 电解质为0.1 mol l -1 所有伏安法测试中的 KOH。
结果与讨论
三种前体(Fe-TPTZ@KIT-MS、Fe-TPTZ 和 TPTZ)的热重分析(TGA)首先显示在图 2a 中。可以看出,当温度超过 300 °C 后,TPTZ 配体的热分解速度很快,仅能节省约 9.0% 的残留质量。然而,在 Fe-TPTZ 配合物的 TGA 曲线上,残余质量约为 40.3%,表明形成了相对稳定的 Fe 2+ 配位相互作用 离子和氮原子,例如 TPTZ 配体中边缘的吡啶氮原子或芳环氮原子 [28]。这种分子级的络合效应主要可以提高Fe-TPTZ配合物的热稳定性,也有助于产生桥连和交联的TPTZ分子。由于使用 KIT-MS 纳米通道的空间限制作用作为新型纳米反应器,Fe-TPTZ 的分解行为可以进一步延迟,导致 Fe-TPTZ@KIT-的最大残留质量(47.4%)多发性硬化症。这种作用有利于高温热解过程中氮掺杂效率和活性位点密度的增加,有利于优化介孔 Fe/N/C 催化剂的 ORR 性能。为了进一步检查稳定的配位相互作用是否完全形成,我们首先通过高分辨率 N1s XPS 光谱表征了 TPTZ、Fe-TPTZ 和 Fe-TPTZ@KIT-MS(图 2b)。显然,吡啶-氮的结合能(B.E.)从TPTZ配体的398.8 eV正移至Fe-TPTZ复合物或Fe-TPTZ@KIT-MS前体的399.1 eV。为Fe 2+ 后吡啶-N原子周围电子密度降低提供了直接证据 离子被引入,因为 B.E.可归因于 Fe 2+ 之间发生的络合相互作用 TPTZ分子边缘上的离子和吡啶-N原子,其中Fe 2+ 的3d未占据轨道 离子可以被吡啶氮原子的孤对电子有效填充。 Fe-TPTZ配合物的形成可以进一步诱导更多的吡啶-氮原子聚集在Fe原子周围,有利于产生更多的Fe-Nx 活性位点结构导致所制备的介孔结构 Fe/N/C 催化剂的 ORR 电催化活性增强。
<图片>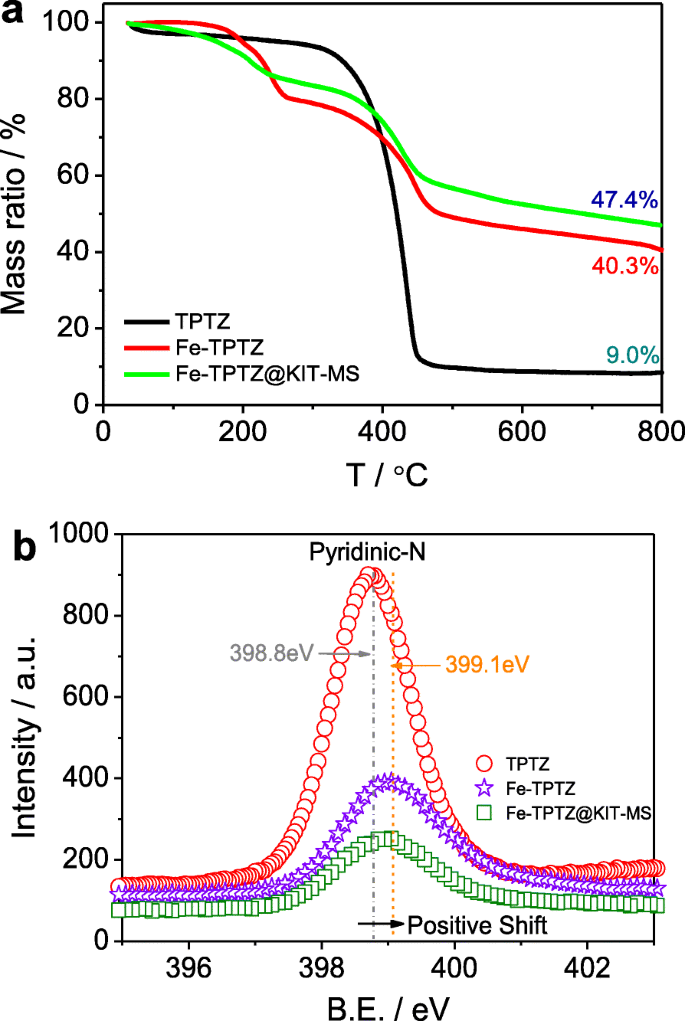
一 TGA 曲线和 b 高分辨率 N1s TPTZ、Fe-TPTZ和Fe-TPTZ@KIT-MS前驱体的XPS光谱
图>基于Fe/N/C型电催化剂的电导特性与其ORR催化性能保持密切联系,我们测量了m的电子电导率 -Fe/N/C-800, m -Fe/N/C-900 和 m -Fe/N/C-950 通过电化学阻抗谱 (EIS)。 EIS数据是在由0.1 M KCl作为电解质和1 mM [Fe(CN)6] 3- 组成的混合溶液中获得的 /[Fe(CN)6] 4- 作为氧化还原探针。 Bode 结果如图 3a 所示,奈奎斯特图如图 3a 的插图所示。奈奎斯特图由具有 R 的五个分量的等效电路拟合(参见附加文件 1:图 S1) s, C dl, R p, R int 和 C ϕ,其中 R s 表示参比电极和工作电极之间发生的电解液电阻。 C dl 表示电极固体/电解质界面上的双电层电容,C ϕ 是与表面中间体形成相关的电荷弛豫,R p 表示 ORR 期间的电荷转移电阻,R int 代表易于形成中间体。 R 的总和 p 和 R int 与 ORR 率有关。附加文件 1:表 S1 中建议了拟合结果。可以发现 R 的总和 p 和 R 对于 m,int 仅为 2232.2 Ω -Fe/N/C-900,但对于 m 约为 2475.5 Ω -Fe/N/C-800 和 m 高达 4418.6 Ω -Fe/N/C-950,分别。较小的总和表明相对较快的 ORR 率,这意味着相应的催化剂 (m -Fe/N/C-900)表现出更好的ORR电催化活性。
<图片>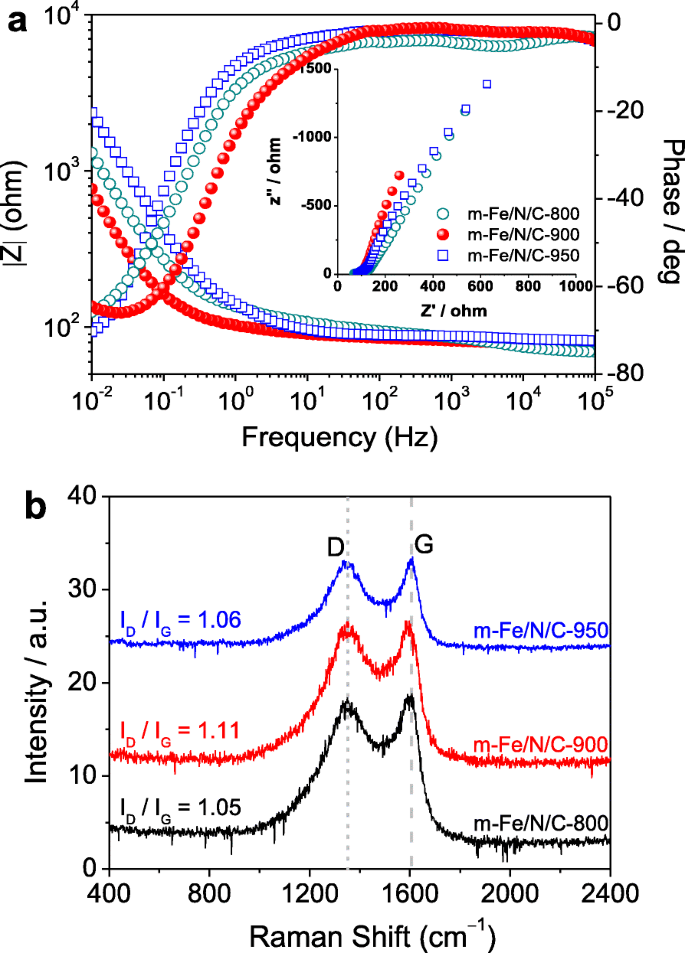
一 m-Fe/N/C-800、m-Fe/N/C-900和m-Fe/N/C-950的EIS波特图;插图是相应的奈奎斯特图。 b m-Fe/N/C-800、m-Fe/N/C-900和m-Fe/N/C-950的拉曼光谱
图>m 的拉曼光谱 -Fe/N/C-800, m -Fe/N/C-900 和 m -Fe/N/C-950(见图3b)被测量,然后被拟合成两个洛伦兹峰。峰值集中在~1350 cm -1 对应于无序结构诱导的 D 带,这通常是由于各种面内杂原子掺杂缺陷的发生 [18]。峰值集中在~1600 cm -1 代表所有石墨结构诱导的 G 带,具有面内 E 2g 振动模式 [25]。它们的强度比 (I D/我 G) 显着用于区分无序度和石墨化程度。发现I D/我 m 上的 G 值 -Fe/N/C-900约为1.11,明显高于m -Fe/N/C-800 (I D/我 G =1.05) 或 m -Fe/N/C-950 (I D/我 G =1.06)。这意味着在m中可以存在更多的含氮缺陷结构和更高的氮原子掺杂含量 -Fe/N/C-900,与XPS分析结果一致。在这项研究中,我们将 Fe-TPTZ 复合物直接固定到分子筛的纳米通道中,因为新的纳米限制反应器可以显着保护它们免受热解分解。此外,Fe 2+ 之间的强分子级配位效应 TPTZ配体中的离子和氮原子也可以在一定程度上稳定Fe-TPTZ前驱体,进一步促进热解过程中的总N含量和N掺杂效率。一些先前报道的结果表明,N 原子的掺杂含量可以对掺杂碳催化剂的电导率产生积极影响 [22, 26],充分支持 m<的最佳电导特性和最高 N 掺杂效率。 /i> -Fe/N/C-900电催化剂的制备。
X射线光电子能谱(XPS)分析用于研究m表面的电子结构和化学成分 -Fe/N/C-800, m -Fe/N/C-900 和 m -Fe/N/C-950。根据调查的 XPS 光谱(图 4 和附加文件 1:图 S2),我们发现所有 Fe/N/C 型催化剂都含有 C、N、O 和 Fe 元素,证明 Fe 和 N原子被成功地掺杂到碳结构中。 XPS 表面分析中 Fe、C 和 N 的总含量以及所制备的介孔 Fe/N/C 型 ORR 电催化剂中的所有 N/C 比率总结在表 1 中。 米 -Fe/N/C-900高达10.3,明显大于m -Fe/N/C-800 (~ 10.0) 或 m -Fe/N/C-950 (~ 4.4),进一步表明 m 中最高的氮掺杂效率和总氮含量 -Fe/N/C-900。 C1s m 的 XPS 分析 -Fe/N/C-900(图 4b)由于与 sp 2 对应的特征峰的出现,也证实了氮原子的掺杂 C=N 键的结合能 (B.E.) 约为 286.5 eV。此外,所制备的 Fe/N/C 型电催化剂的 XPS 光谱中 Fe 元素的信号相对较弱,因为在酸性溶液中浸出后 Fe 含量非常低。为了检查 Fe 原子的电子态,我们分析了 Fe 2p m 的 XPS 谱 图 2b 中的 -Fe/N/C-900,用 B.E. 拟合成四个峰。分别为 710.3、712.9、716.8 和 725.3 eV。位于~710.3 eV的峰可以归因于Fe-N键的特征峰,表明Fe 2+ 的出现 m 中的状态 -Fe/N/C-900 [29, 30]。该会。位于约 712.9 eV 和 725.3 eV 对应于 Fe 3+ 2p 3/2 和 Fe 3+ 2p 分别为 1/2(图 4c),这表明 Fe-TPTZ 复合物中的 Fe 原子可以被部分氧化;然而,位于 716.8 eV 的峰可以代表 Fe-N 键的卫星峰。为了进一步确定 Fe 氧化物的化学状态,我们分析了 O1s m 的频谱 -Fe/N/C-900(图 4d)。它用 B.E. 分为三个峰。 531.0、532.3 和 533.2 eV,分别对应于 C–O–C、C=O 和 Fe–O [31]。上述结果可能表明,Fe原子的存在状态主要由m中的Fe-N和Fe-O键组成。 -Fe/N/C-900电催化剂。
<图片>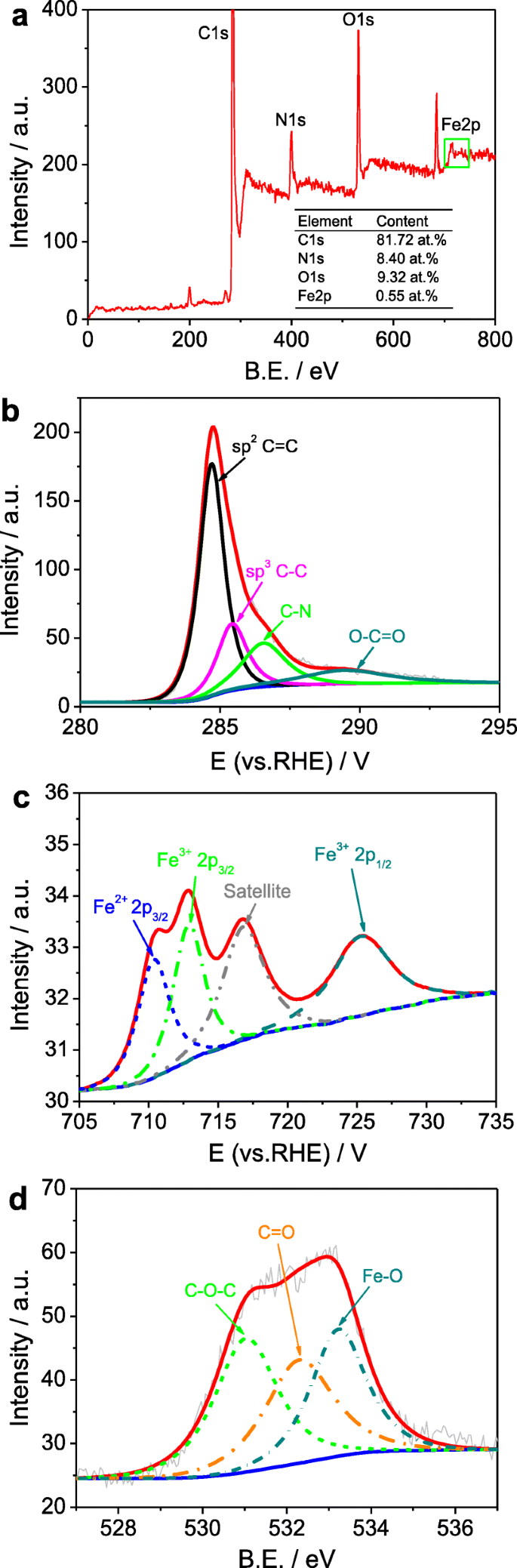
调查 (a ), Fe 2p (b ), O 1s (c ), 和 C 1s (d ) m 的 XPS 光谱 -Fe/N/C-900
图> 图>拟合的 N1s m 的 XPS 光谱 -Fe/N/C-800 和 m -Fe/N/C-900(参见图 5a、b)显示了四个 B.E. 398.3、399.5、401.1 和 406.1 eV,这分别归因于吡啶-N、Fe-N、石墨-N 和氧化-N (-NO2) [10, 16, 25, 26, 32, 33] .然而,拟合的 N1s m 的 XPS 谱 -Fe/N/C-950(图 5c)只显示了三个 B.E. 399.2、401.4 和 406.4 eV,分别对应于 Fe-N、石墨-N 和氧化-N (-NO2)。注意到由于氧原子的电负性更强,氮原子的电子云密度发生了移动,导致氧化峰出现在高能阶段。石墨-N基团的相对比例在总氮含量中占主导地位,但随着热解温度的增加,惰性氧化-N(-NO2)的相对比例从17.8增加到38.7 at.%,如图5d所示.此外,较高的热解温度会导致m中吡啶-N基团的消失。 -Fe/N/C-950。上述结果表明,Fe-N 基团可以在所制备的 Fe/N/C-型催化剂中形成,但活性吡啶-和石墨-N 基团对确定 Fe/N/C- 的 ORR 活性具有重要意义。型电催化剂。
<图片>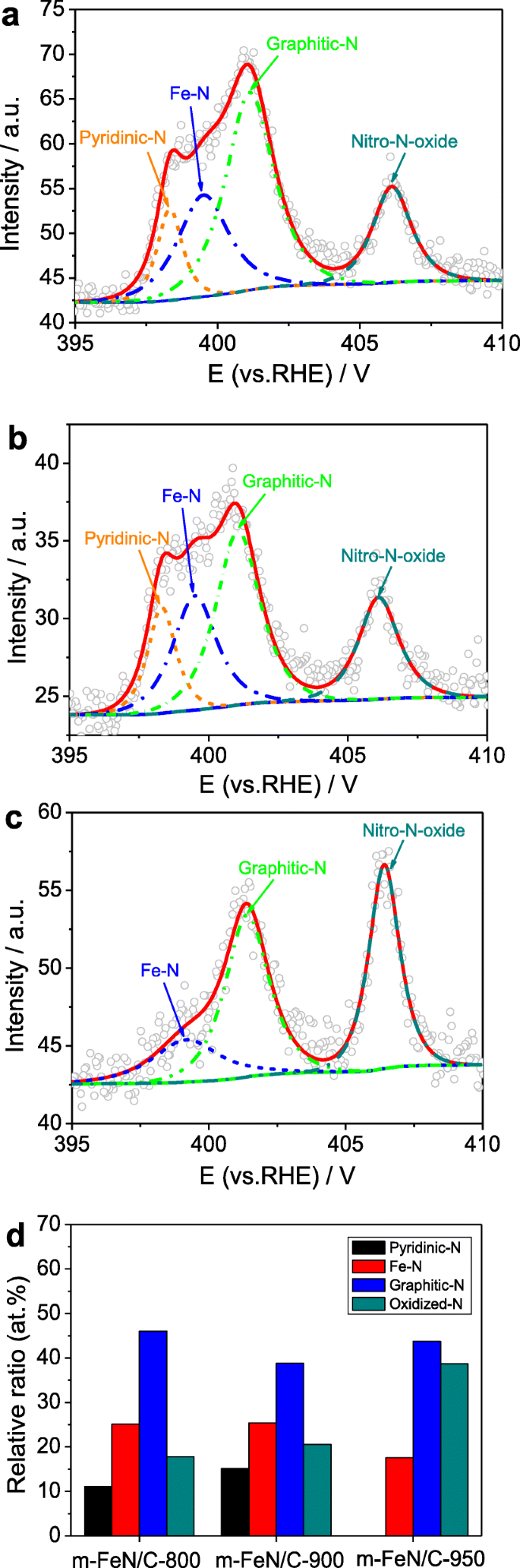
N 1s m 的 XPS 光谱 -Fe/N/C-800 (a ), m -Fe/N/C-900 (b ), m -Fe/N/C-950 (c ), 以及它们在总 N 含量中的相对含量 (d )
图>m的形态分析 -Fe/N/C-900 催化剂如图 6 和附加文件 1:图 S3 所示。 m 可以存在大量海绵状的形状 -Fe/N/C-900(图 6a、b)通过金属有机物 (Fe-TPTZ) 复合物的高温热解合成,受限地固定在分子筛的纳米通道中。高分辨率 TEM 图像(图 6c、d)显着显示 m 内部有许多高度有序的介孔结构 -Fe/N/C-900,这在很大程度上可以源自作为纳米通道限制反应器的分子筛的去除。此外,在图 6e 中可以清楚地看到边缘上的无序碳结构和几个介孔,这是由于氮原子的掺杂。因此,m 的多孔特性和布鲁诺-埃米特-特勒 (BET) 表面积 -Fe/N/C-900 还通过氮吸附/解吸等温线进行了研究(图 6f 的插图)。可以观察到 Langmuir IV 型等温线,表明 m 具有高度介孔特性 -Fe/N/C-900 电催化剂。 m的BJH孔径分布可以进一步证实 -Fe/N/C-900(图 6f),显示出高 BET 表面积(A BET ~ 1035 m 2 g -1 ) 和总孔体积 (V 总计 ~ 1.22 cm 3 g -1 ) 平均孔径 (D p) 约为 4.7 nm。所展示的两个最大孔径(4.3 和 9.3 nm)归因于 m 中介孔的最大位置 -Fe/N/C-900。这些介孔可以为电解质、反应物和产物的快速运输提供更方便的纳米通道;降低氧分子向掺氮活性位点的传输阻力;并提高m的ORR催化性能 -Fe/N/C-900。此外,使用分子筛的纳米通道作为纳米限域反应器有利于生产具有超高表面积的海绵状三维介孔碳,有利于增强催化 ORR 活性位点的暴露。元素映射图像(图 7)显示了四种主要元素(Fe、N、C 和 O)在 m 表面的均匀分布 -Fe/N/C-900,这在一定程度上可能是分子筛的纳米通道限域效应和Fe-TPTZ配合物的稳定分子配位效应的协同作用所致。
<图片>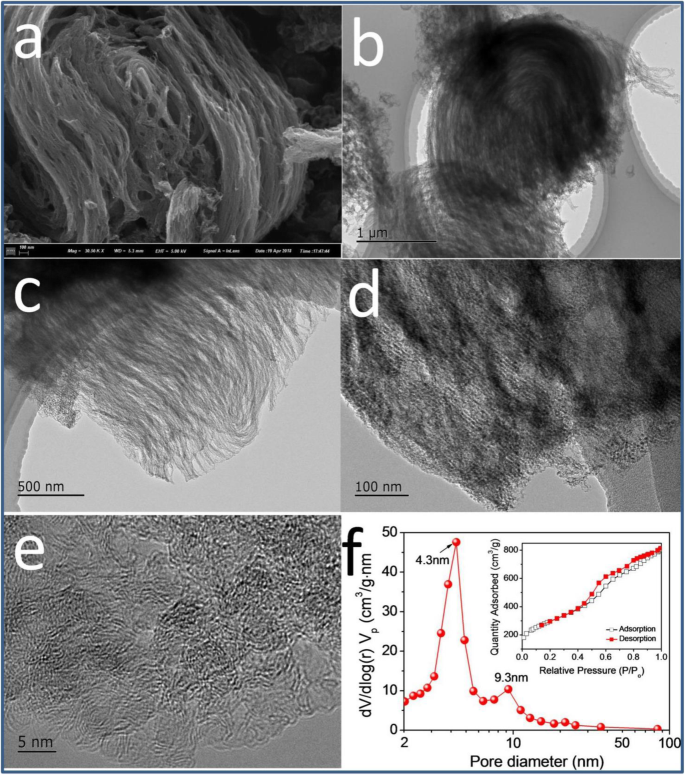
SEM (a ) 和高分辨率 TEM 图像 (b –e ) m -Fe/N/C-900; f m的孔径分布 -Fe/N/C-900;插图为氮气吸附-解吸等温线
图> <图片>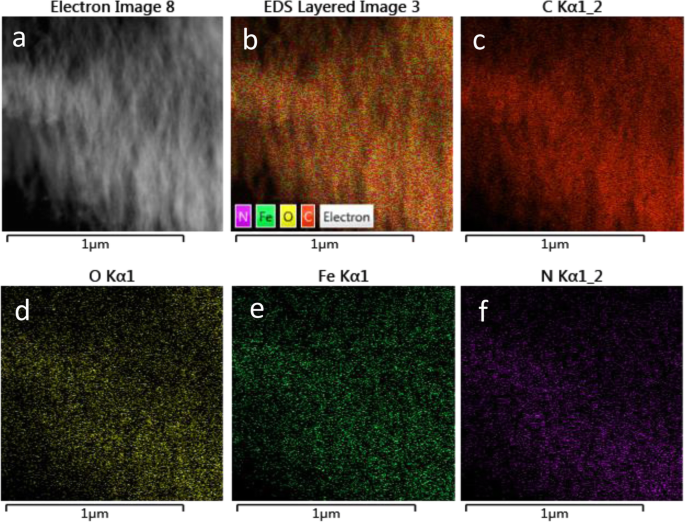
TEM 图像 (a ) m -Fe/N/C-900 和相应的 C、O、Fe 和 N 元素映射图像 (b –f )
图>通过循环伏安图 (CV) 或线性扫描伏安图 (LSV) 测试所有制备的催化剂对 ORR 的电催化活性。图 8a 显示了 m 的 CV -Fe/N/C-900 in N2 vs O2-saturated 0.1 mol l -1 KOH 电解液。在 O2 饱和电解质中,ORR 峰值出现在 0.86 V vs. RHE 处;然而,在 N2 饱和电解质中观察到无特征的 CV 曲线,表明 m 的 ORR 催化活性 -Fe/N/C-900 具有起始电位 (E 起始)1.0 V。此外,热处理温度(800-950 °C)对ORR性能的影响在图8b中进行了研究。根据最大峰值电流密度(j p),以及最正的峰值电位 (E p) 可以在 m 处获得 -Fe/N/C-900。 m 的 ORR 活性 -Fe/N/C-900 可以与其他报道的掺杂碳催化剂相媲美(参见附加文件 1:表 S2)。为了深入了解 Fe/N/C 型电催化剂的 ORR 动力学行为,我们通过 LSV 方法结合 RRDE 进一步测试了 ORR 极化曲线,如图 8c 所示。根据 RRDE 数据,转移的电子数 (n ) 和 ORR 期间的 H2O2 产率 (H2O2%) 是通过使用以下方程估计的。分别为 (1) 和 (2)。计算公式如下[34]:
$$ \%H{O}_2^{-}=100\times \frac{2{I}_r/N}{I_d+\left({I}_r/N\right)} $$ (1) $$ n=4\times \frac{I_d}{I_d+{I}_r/N} $$ (2)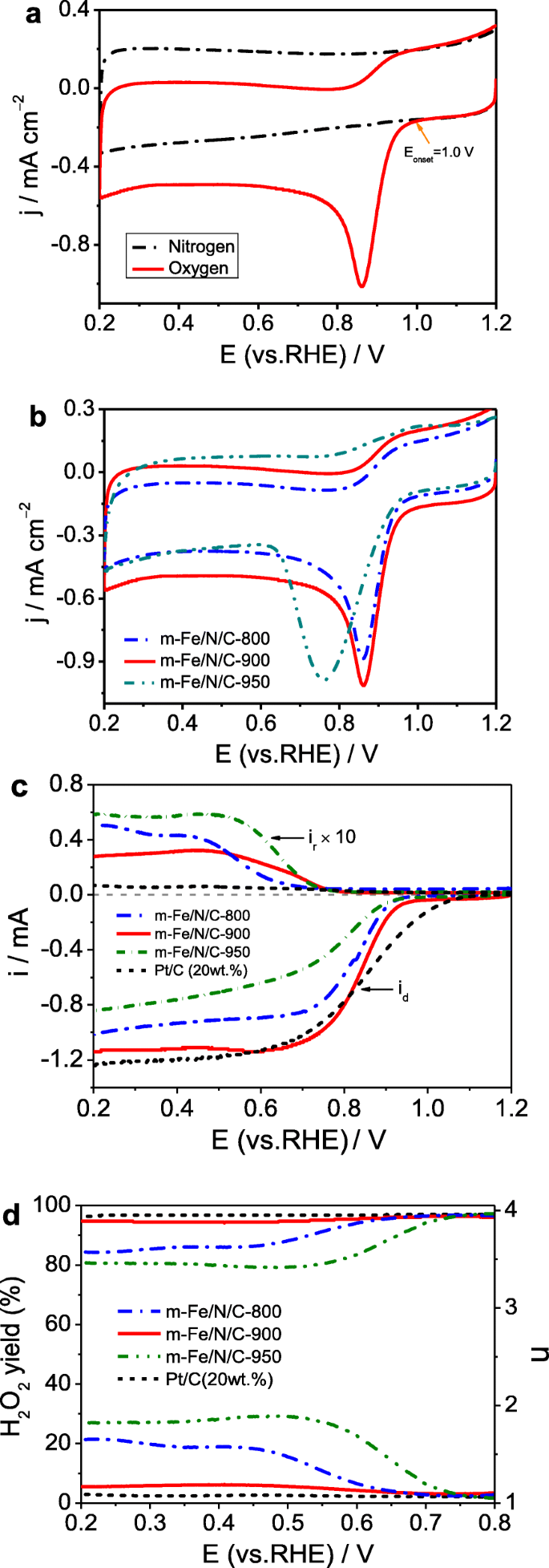
一 m的CV曲线 -Fe/N/C-900 在 N2 与 O2 饱和的 0.1 M KOH 溶液中。 b m的CV曲线 -Fe/N/C-800, m -Fe/N/C-900 和 m -Fe/N/C-950 in O2-saturated 0.1 mol l -1 氢氧化钾溶液。 c 在 m 的 RRDE 上使用 LSV 获得的盘和环电流 -Fe/N/C-800, m -Fe/N/C-900 和 m -Fe/N/C-950 in O2-saturated 0.1 mol l -1 氢氧化钾溶液。 d m对应的电子转移数和H2O2产率 -Fe/N/C-800, m -Fe/N/C-900, and m -Fe/N/C-950 derived from c
图>其中我 d is the Faradaic disk-current, I r is the Faradaic ring-current, and N is the collection efficiency of ring electrode (0.38). The Pt-ring potential was set at 1.5 V (vs. RHE) as reported elsewhere. Figure 8d displays the relatively calculated results. The H2O2 yield (<6.5%) and n value (3.88–3.94) are obtained on m -Fe/N/C-900, dominating a four-electron ORR pathway. It suggests that this catalyst is a valuable substitute for the traditional 20 wt.% Pt/C catalyst (purchased from Aladdin Industrial Co. Ltd.), although the H2O2 yield on m -Fe/N/C-900 is slightly higher. In addition, the half-wave potential (E 1/2) for ORR of m -Fe/N/C-900 is about 0.841 V approaching that of 20 wt.% Pt/C (~ 0.848 V), and the limited current density (j d) of m -Fe/N/C-900 is almost identical to that of 20 wt.% Pt/C. Compared to the m -Fe/N/C-900 catalyst, higher H2O2 yield and smaller n value can be gained on both m -Fe/N/C-800 and m -Fe/N/C-950, but the electron transfer number on both m -Fe/N/C-800 and m -Fe/N/C-950 still belongs to the range (3.4–4.0), showing that the ORR on two Fe/N/C-type happens with a mixed process of two and four-electron transfer pathways. The above results further approve that the m -Fe/N/C-900 synthesized by nanochannel-confined control of the pyrolysis process to improve the nitrogen-doping efficiency and increase the nitrogen-doped active-site density has exhibited the optimal ORR catalytic performance in alkaline electrolyte.
We also discuss the effect of different transition metals on the ORR catalytic activity of Fe/N/C-type electrocatalysts. The obtained LSV curves on the RRDE are indicated in Fig. 9a, and the corresponding n value and H2O2 yield are demonstrated in Fig. 9b. E 1/2 values are about 0.785 V for m -Cu/N/C-900 and 0.780 V for m -Ni/N/C-900, respectively, which are lower compared to the m -Fe/N/C-900. The j d follows the order of m -Fe/N/C-900> m -Cu/N/C-900> m -Ni/N/C-900, further suggesting the best ORR catalytic activity of m -Fe/N/C-900 in 0.1 mol l -1 KOH solution. Compared to the m -Fe/N/C-900 catalyst, higher H2O2 yield and smaller n value are obtained on both m -Cu/N/C-900 and m -Ni/N/C-900. What is noteworthy is that the H2O2 yield on m -Cu/N/C-900 and m -Ni/N/C-900 is over twice as large as that on m -Fe/N/C-900. However, the n value on both m -Cu/N/C-900 and m -Ni/N/C-900 is 3.5–4.0, indicating that the ORR process on two Fe/N/C-type electrocatalysts follows a two- and four-electron mixed transfer pathway but is dominant in a four-electron reaction pathway. Besides, the electrochemical long-term stability for ORR catalysis of m -Fe/N/C-900 is of great significance in the practical applications. An accelerated aging test (AAT) was carried out by successive CV scanning tests from 0.2 to 1.2 V vs RHE for 5000 cycles at 200 mV s -1 in oxygen-saturated 0.1 M KOH electrolyte. The ORR electrocatalytic behavior of m -Fe/N/C-900 has been further evaluated under the same conditions as above experiments. CV curves for ORR activity of m -Fe/N/C-900 before and after doing the AAT are almost unchanged on the E p (~ 0.86 V), but the j p is slightly reduced (Fig. 9c). LSV curves of m -Fe/N/C-900 (Fig. 9d) also reveal an only ~ 12 mV negative shift in the E 1/2 and a negligible decrease in the j d. However, the commercial Pt/C (20 wt.% Pt) catalyst after doing the AAT has exhibited about 55 mV of the negative shift in E 1/2 and an obvious reduction in the j d (Fig. 9d). Results show that the m -Fe/N/C-900 has more excellent electrocatalytic stability compared to the Pt/C catalyst, further suggesting that it is a valuable and promising substitute for the conventional Pt-based materials in alkaline electrolytes.
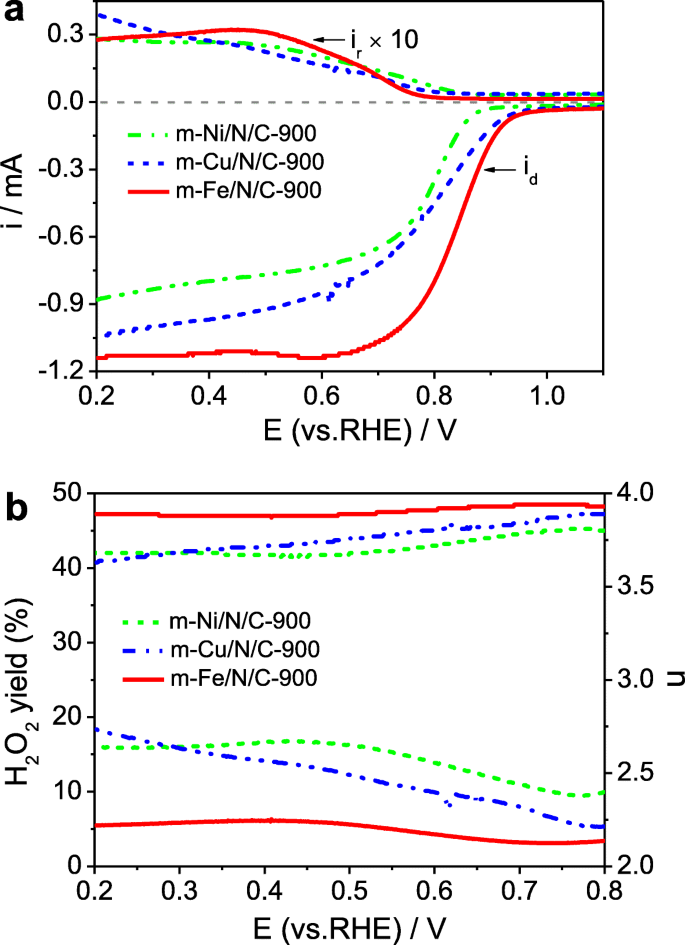
一 Disk and ring currents obtained with LSVs on RRDE for m -Ni/N/C-900, m -Cu/N/C-900, and m -Fe/N/C-900 in O2-saturated 0.1 mol l -1 KOH solution. b The corresponding electron transfer number and H2O2 yield of m -Ni/N/C-900, m -Cu/N/C-900, and m -Fe/N/C-900 derived from a . c CV curves and d LSV curves of m -Fe/N/C-900 before and after continuous scanning for 5000 cycles in O2-saturated 0.1 M KOH solution
图>In order to discuss the catalytically active sites of Fe/N/C-type catalysts and study the role of the molecular coordination and nanochannel-confined effects, we have further examined the N/C-900, Fe/N/C-900, and 20 wt.% Pt/C catalysts for comparison of the ORR catalytic behavior. The tested results of the ORR activity are indicated in Fig. 10a. The onset potentials of ORR are about 0.683 V for N/C-900 and 0.740 V for Fe/N/C-900, being largely lower than those of m -Fe/N/C-900 (0.841 V) and 20 wt.% Pt/C catalysts (0.848 V). Figure 10b shows the corresponding H2O2 yields and transferred electron numbers in the ORR process. Given other Fe/N/C-type catalysts and the Pt/C catalyst, the H2O2 yield on N/C-900 is the highest and the transferred electron number on N/C-900 is the smallest, suggesting the worst ORR catalytic activity of N/C-900. In addition, the H2O2 yield on Fe/N/C-900 is mainly higher than that on m -Fe/N/C-900 and the transferred electron number on Fe/N/C-900 is far lower than that on m -Fe/N/C-900 in the same range (0.2–0.8 V vs RHE), indicating a relatively inferior ORR activity. Thus, it can be concluded that the ORR performance complies with the sequence of Pt/C> m -Fe/N/C-900> Fe/N/C-900> N/C-900. These results show that the formation of Fe-TPTZ compounds with the strong molecular-level coordination effect is beneficial to produce the Fe/N/C-type catalysts with high ORR activity, and the utilization of the nanochannel-confined effect can reduce the decomposition speed of Fe-TPTZ compounds and protect the nitrogen-rich active sites (e.g., Fe–N, graphitic-N, or pyridinic-N) from the thermal loss during the pyrolysis process (see the TG analysis, Fig. 2a), which can enhance the ORR performance of Fe/N/C-type catalysts in alkaline medium.
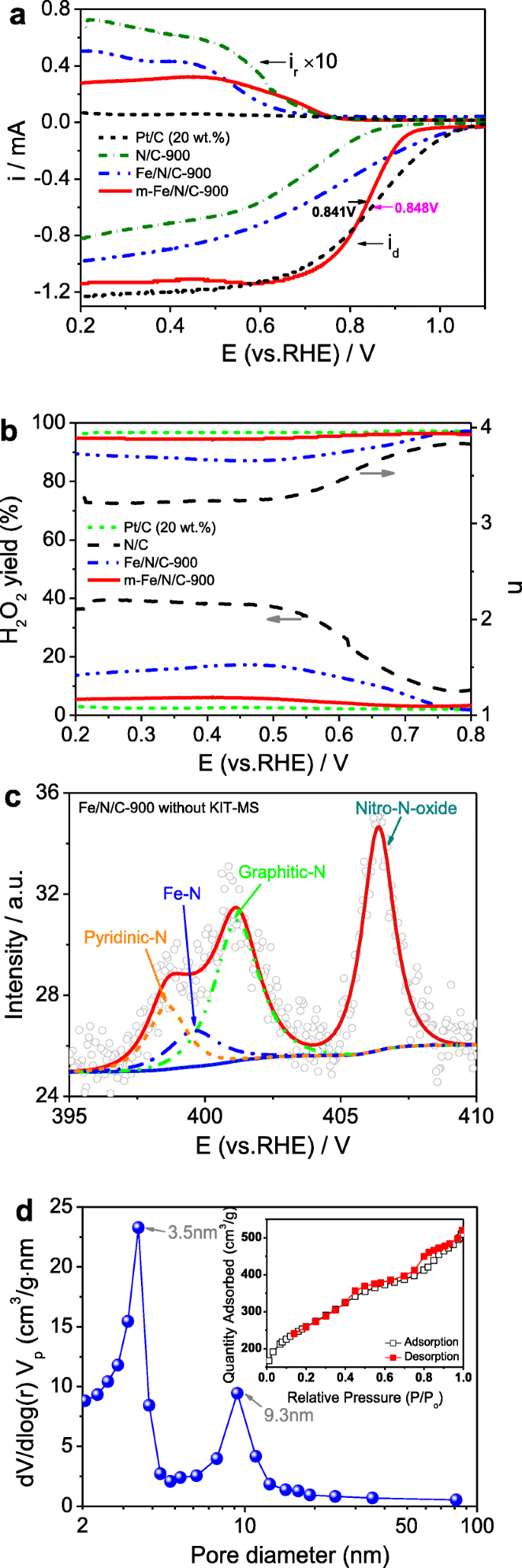
一 Disk and ring currents obtained with LSVs on RRDE for N/C-900, Fe/N/C-900, m -Fe/N/C-900, and Pt/C (20 wt.%) in O2-saturated 0.1 mol l -1 KOH solution. b The corresponding electron transfer number and H2O2 yield of N/C-900, Fe/N/C-900 m -Fe/N/C-900, and Pt/C (20 wt.%) derived from a
图>To deepen the understanding of active sites and their ORR catalysis mechanism, we also characterized the prepared Fe/N/C-type catalysts by the spectra of X-ray diffraction (XRD) and X-ray photoelectric spectroscopy (see Additional file 1:Figures S4 and S5). The XRD data display that the density of carbon (002) peak follows the sequence of N/C-900> Fe/N/C-900> m -Fe/N/C-900, and the positions for carbon (002) peak in three catalysts are negatively shifted because of the production of more sp 2 C–N groups into the graphitic layers and the decrease of graphitization. It also implies that the different N content may be doped into the carbon skeleton in the catalyst, and both N-doping efficiency and N content can follow the similar order with their ORR activity. More significantly, we further compare the structural and porous differences between Fe/N/C-900 and m -Fe/N/C-900 to better study the nanochannel-confined effect of molecular sieves. The tested XPS survey spectrum of Fe/N/C-900 was indicated in Additional file 1:Figure S5, and its surface contents of Fe, C, and N and the N/C ratio were summarized in Table 1. The Fe content and N content are ~ 0.41 at.% and ~ 4.48 at.%, but the N/C ratio is only 5.1 in the Fe/N/C-900, being lower compared to the m -Fe/N/C-900. It suggests the N-doping efficiency was improved by introducing the nanochannel-confined protection strategy into the carbonization process, which can prove our key views of this work. Besides, the fitted N1s XPS spectrum of Fe/N/C-900 is indicated in Fig. 10c. It displays the existence of four peaks with B.E. of 398.6, 399.6, 401.2, and 406.4 eV, which still correspond to the pyridinic-N, Fe–N, graphitic-N, and oxidized-N (–NO2) with a relative percentage of 14.0, 10.2, 35.4, and 40.4 at.%, respectively. Compared with the m -Fe/N/C-900, the total ratio of active N-rich groups such as pyridinic-N, Fe–N, and graphitic-N obviously decreases about 19.8 at.%; however, the relative ratio of Fe–N groups is reduced about 15.2 at.%. Thus, associating with fore-mentioned XPS data and the catalytic activity data, we conclude that the electrocatalytically active sites may be pyridinic- and graphitic-N groups for holding the ORR performance, but the enhancement of the ORR activity may be related to the relative ratio of Fe–N groups for our system. The role of the nanochannel-confined effect cannot only reduce the loss of total N content, but also can largely increase the N-doping efficiency and improve the effective ORR active-site density in the catalyst. Besides, we further analyzed porous characteristic and BET specific surface area of Fe/N/C-900 without the usage of the KIT-MS nanoreactor. The nitrogen adsorption/desorption isotherms with a similar Langmuir IV-type isotherm curve are seen in the inset of Fig. 10d. It suggests that highly mesoporous characteristic is still existed in Fe/N/C-900, supported by the analysis of BJH pore-size distribution (Fig. 10d). A BET (~ 875 m 2 g -1 ) and V total (~ 0.76 cm 3 g -1 ) with an average D P of only ~ 3.5 nm are obtained on the Fe/N/C-900, which are obviously lower than those on the m -Fe/N/C-900. The large difference on pore structures between Fe/N/C-900 and m -Fe/N/C-900 can be derived from the stable coordination effect and the nanochannel-confined role of a KIT-MS reactor in the preparation of m -Fe/N/C-900. It will also influence their inherent ORR performance because higher A BET and V total can help to supply abundant catalytic sites and increase the exposed surface active-site density, being beneficial to the adsorption and electro-reduction process of O2 molecule [35]. Notably, we should pay much attention to the effect of the conductivity characteristic. Generally, a higher conductivity characteristic of m -Fe/N/C-900 corresponds to a relatively faster ORR electron transportation process. Therefore, facile design and control of active nitrogen-rich groups (pyridinic-N, graphitic-N, and Fe–N, etc.) is of great importance to fabricate mesopore-structured Fe/N/C electrocatalysts for the ORR, but further improving the conductance, N-doped active-site density, and mesoporous characteristic is another key issue of concern to obtain the high performance.
结论
In conclusion, here, we propose a new and effective strategy to design a Fe/N/C-type electrocatalyst (m -Fe/N/C-900) with ultrahigh BET surface area (1035 m 2 g -1 ) and total pore volume (1.22 cm 3 g -1 ) via nanochannel-confined high-temperature carbonization of Fe 2+ ions coordinated with 2,4,6-tri(2-pyridyl)-1,3,5-triazine compound as a single-source Fe, N, and C precursor. The elemental mapping images of m -Fe/N/C-900 further prove the homogeneous distribution of Fe, N, C, and O elements on its surface. On the one hand, the strong molecular-coordination role in Fe-TPTZ complex can enhance the thermal stability and stabilize higher contents of Fe–N active sites during pyrolysis process. On the other hand, the utilization of abundant nanochannels of molecular sieve as a novel nanoconfined reactor does not only benefit to produce spongy-like mesoporous carbons with excellent pore structure and conductivity characteristic, but also facilitate to decrease the loss of N atoms and improve the N-doping efficiency and N-doped active-site density, resulting in the ORR activity enhancement. Electrochemical tests indicate the m -Fe/N/C-900 displays unexpected catalytic performance with an ORR half-wave potential of ~ 0.841 V versus RHE and high limited current density approaching the commercial Pt/C catalyst. Additionally, low H2O2 yield (<6.5%) and high electron transfer number (3.88–3.94) on m -Fe/N/C-900, indicating that it is a valuable substitute for the traditional Pt/C catalyst. The comparison analysis of XPS data and electrocatalytic activity data can point out that active pyridinic and graphitic-N groups may be the electrocatalytically ORR-active sites, but the enhancement of the ORR activity may be related to the relative ratio of Fe–N groups for our system. This study provides a new idea or method for the synthesis of high-performance Fe/N/C electrocatalysts via integrating molecular-level coordination and nanochannel-confined effects and does also help the researchers better deepen the understanding of nitrogen-doped active sites and their ORR catalysis mechanism for Fe/N/C-type electrocatalysts to a certain extent. However, what cannot be ignored is that effective improvement and optimization of nitrogen-doping active site density, conductivity, and porous characteristics is essential to boost the ORR electrocatalytic activity.
数据和材料的可用性
The authors declare that the materials and datasets used or analyzed during the current study are available from the corresponding author on reasonable request.
缩写
- AAT:
-
Accelerated aging test
- AE:
-
Auxiliary electrode
- 赌注:
-
布鲁诺-埃米特-特勒
- 简历:
-
循环伏安法
- E 1/2 :
-
Half-wave potential
- E :
-
Peak potential
- Fe/N/C catalyst:
-
Iron/nitrogen/carbon catalyst
- Fe-TPTZ:
-
Fe coordinated with 2,4,6-tri(2-pyridyl)-1,3,5-triazine complexes
- GC:
-
Glassy carbon
- HR-TEM:
-
高分辨透射电子显微镜
- KIT-MS:
-
KIT-6 molecular sieves
- LSV:
-
线性扫描伏安法
- ORR:
-
氧还原反应
- Pt/C:
-
铂/碳催化剂
- RDE:
-
Rotation disk electrode
- RE:
-
Reference electrode
- RHE:
-
可逆氢电极
- RRDE:
-
Rotation ring-disk electrode
- SCE:
-
饱和甘汞电极
- SEM:
-
扫描电镜
- TGA:
-
热重分析
- TPTZ:
-
2,4,6-Tri(2-pyridyl)-1,3,5-triazine
- WE:
-
Working electrode
- XPS:
-
X射线光电子能谱
- XRD:
-
X射线衍射
纳米材料
- 用于合成和生物医学应用的荧光纳米材料的进展和挑战
- Ag 修饰的 SnO2 微球的一锅绿色合成:一种用于还原 4-硝基苯酚的高效且可重复使用的催化剂
- 合成单分散二元 FePt-Fe3O4 纳米粒子的后处理方法
- 用于电催化氧还原反应的重石墨-氮自掺杂高孔隙率碳
- 一维混合二元氧化物 CeO2-LaO x 支持的金催化剂的合成和 CO 氧化活性
- 用于超灵敏葡萄糖传感的介孔氧化镍 (NiO) 纳米瓣
- 桔梗皂苷(桔梗)用于金和银纳米颗粒的绿色合成
- 表面功能化磁性纳米复合材料的简便合成,可有效选择性吸附阳离子染料
- 铂钴纳米催化剂上的氧还原反应:(双)硫酸盐阴离子中毒
- 用于高性能超级电容器的二维 VO2 介孔微阵列
- 腐殖酸辅助合成二硫化钼/碳纳米复合材料通过共沉淀/煅烧途径用于高性能锂离子电池
- 源自静电纺丝和原位热解的高活性和稳定的 Fe-N-C 氧还原电催化剂